INTRODUCTION

MATERIALS AND METHODS
Animals and study design
Table 1
Compositions of the experimental diets
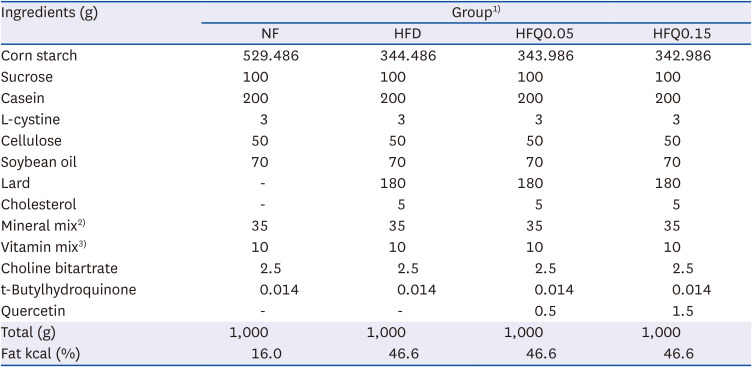
Sample preparation
Glycerol-3-phosphate dehydrogenase (GPDH) activity in adipose tissue
mRNA expressions of transcription factors related to adipocyte differentiation in liver
Table 2
PCR primer sequences of transcription factors related to adipocyte differentiation and MMPs

mRNA expressions and concentrations of MMPs in adipose tissues
Statistical analysis

RESULTS
Body weights, food intake, and organ weights
![]() | Fig. 1Body weight changes in the experimental groups.Lines represent mean weights ± SEs of the experimental groups. Five-wk-old C57BL/6J mice (Daehan Biolink) were randomly allocated to 4 experimental groups: normal fat diet (ND); high fat diet (HFD); high fat diet + quercetin 0.05% (HFQ0.05); and high fat diet + quercetin 0.15% (HFQ0.15).
NS, no significant intergroup difference.
Different symbols above lines for each wk indicate significant intergroup differences at P < 0.05 as determined by Duncans multiple range test.
|
Table 3
Body weights, body weight gains, dietary intakes, and FER of the experimental groups

Table 4
Mean organ weights in the experimental groups (g)

GPDH activity assay
![]() | Fig. 2Effect of 16 weeks of quercetin administration on GPDH activity in adipose tissues.Bars represent means ± SEs of the experimental groups. Five-wk-old C57BL/6J mice (Daehan Biolink) were randomly allocated to 4 experimental groups:normal fat diet (ND); high fat diet (HFD); high fat diet + quercetin 0.05% (HFQ0.05); and high fat diet + quercetin 0.15% (HFQ0.15).
GPDH, glycerol-3-phosphate dehydrogenase.
Different letters above bars indicate significant intergroup differences at P < 0.05 as determined by Duncans multiple range test.
|
mRNA expressions of transcription factors related to adipocyte differentiation
![]() | Fig. 3Effects of 16 weeks of quercetin administration on the mRNA expressions of transcription factors related to adipocyte differentiation in the liver.Total RNA was isolated using TRI-Reagent, and cDNA was synthesized using SuperScript II reverse transcriptase from total RNA. Real-time PCR with SYBR Green was performed using standard procedures to assess the mRNA expressions in liver samples obtained from each group. β-Actin levels were used to ensure equal loadings. Applied Biosystems StepOne software v2.1 was used. Bars represent means ± SEs of the experimental groups. Five-wk-old C57BL/6J mice (Daehan Biolink) were randomly allocated to 4 experimental groups: normal fat diet (ND); high fat diet (HFD); high fat diet + quercetin 0.05% (HFQ0.05); and high fat diet + quercetin 0.15% (HFQ0.15).
PCR, polymerase chain reaction; C/EBPβ, CCAT/enhancer-binding protein β; C/EBPα, CCAT/enhancer-binding protein α; PPARγ, peroxisome proliferator activated receptor γ; FABP4, fatty acid binding protein 4.
Different letters above bars indicate significant intergroup differences (P < 0.05 as determined by Duncans multiple range test).
|
mRNA expressions and concentrations of MMPs
![]() | Fig. 4Effects of 16 weeks of quercetin administration on mRNA expression, concentrations of MMP-2 in adipose tissues.To measure the mRNA expression of MMP-2, total RNA was isolated using TRI-Reagent, and cDNA was synthesized using total RNA and SuperScript II reverse transcriptase. Real-time PCR with SYBR Green was performed using standard procedures to assess mRNA expressions in epididymal fat pad samples. (A) β-Actin levels were used to ensure equal loadings. Applied Biosystems StepOne software v2.1 was used. (B) MMP-2 protein levels were measured using the MMP-2 ELISA kit (MBS722437, MyBioSouce. (C) MMP-2 activity was measured using the MMP-2 Biotrack activity assay system kit (RPN 2631; GE Healthcare. Bars represent means ± SEs of the experimental groups. Five-wk-old C57BL/6J mice (Daehan Biolink) were randomly allocated to 4 experimental groups: normal fat diet (ND); high fat diet (HFD); high fat diet + quercetin 0.05% (HFQ0.05); and high fat diet + quercetin 0.15% (HFQ0.15).
MMP, matrix metalloproteinase.
Different letters above bars indicate significant intergroup differences (P < 0.05 as determined by Duncans multiple range test).
|
![]() | Fig. 5Effects of 16 weeks of quercetin administration on the mRNA expression, concentrations of MMP-9 in adipose tissues.To measure the mRNA expression of MMP-9, total RNA was isolated using TRI-Reagent, and cDNA was synthesized using total RNA with SuperScript II reverse transcriptase. Real-time PCR with SYBR Green was performed using standard procedures to determine mRNA expressions in epididymal fat pad samples. (A) β-Actin levels were used to ensure equal loadings. Applied Biosystems StepOne software v2.1 was used. (B) MMP-9 levels were measured using the MMP-9 ELISA kit (MBS720876, MyBioSource, and (C) MMP-9 activities were measured using an MMP-9 activity assay kit (QuickZyme BioScience). Bars represent means ± SEs of the experimental groups. Five-wk-old C57BL/6J mice (Daehan Biolink) were randomly allocated to 4 experimental groups: normal fat diet (ND); high fat diet (HFD); high fat diet + quercetin 0.05% (HFQ0.05); and high fat diet + quercetin 0.15% (HFQ0.15).
MMP, matrix metalloproteinase; PCR, polymerase chain reaction; ELISA, enzyme-linked immunosorbent assay.
Different letters above bars indicate significant intergroup differences at P < 0.05 as determined by Duncans multiple range tests.
|

DISCUSSION
