RESULTS
Body weights
With similar initial body weight (C, 73.39 ± 2.60 g; M50, 73.66 ± 2.13 g; M62.5, 73.60 ± 2.02 g; M75, 73.43 ± 1.81 g; M100, 73.47 ± 1.80 g; P > 0.05), after 14 days of feeding, no significant differences of final body weight (C, 167.25 ± 4.75 g; M50, 168.78 ± 3.78 g; M62.5, 169.02 ± 2.70 g; M75, 169.03 ± 7.51 g; M100, 171.24 ± 2.52 g; P > 0.05), and body weight (C, 93.86/14 days ± 3.79 g; M50, 95.12/14 days ± 3.12 g; M62.5, 95.42 ± 4.78 g; M75, 95.60 ± 4.16 g; M100, 97.77 ± 3.48 g; P > 0.05) were found among the experimental groups.
Hepatic HNE contents
After oral administration for 14 days, the hepatic contents of HNE decreased with increasing
L-methionine levels. As shown in
Fig. 2, compared to C, groups M50, M62.5, M75 and M100 showed 12.92% (
P < 0.05), 14.92% (
P < 0.05), 17.75 (
P < 0.05), and 20.23% (
P < 0.05) less hepatic HNE accumulation in growing rats, respectively, showing a good dose-dependent relationship. The significant negative correlation between
L-methionine intake and hepatic HNE content (r = −0.8171,
P < 0.05) suggested that
L-methionine could suppress lipid peroxidation.
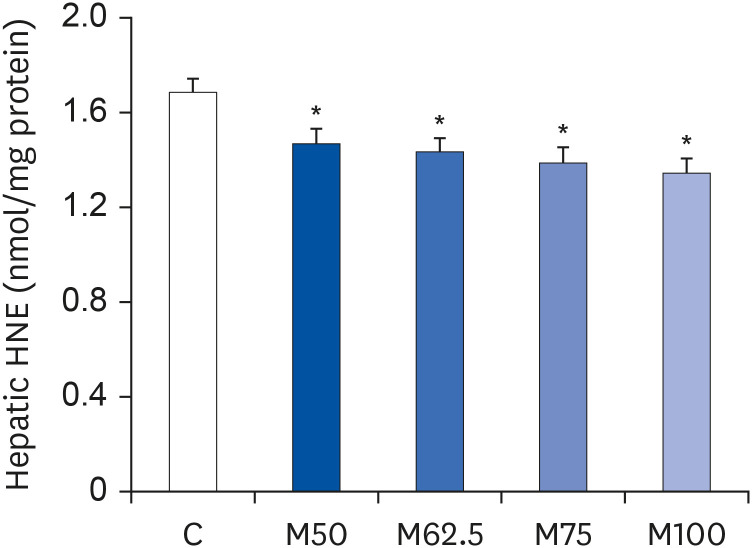 | Fig. 2
Hepatic contents of HNE in growing rats. Hepatic contents of HNE in growing rats after the oral administration of L-methionine for 14 days. The values are expressed as the means ± SEM (n = 6).
HNE, 4-hydroxy-2-nonenal; C, control; M50, basal diet and administered L-methionine orally at 215.0 mg/kg bw; M62.5, basal diet and administered L-methionine orally at 268.8 mg/kg bw; M75, basal diet and administered L-methionine orally at 322.5 mg/kg bw; M100, basal diet and administered L-methionine orally at 430.0 mg/kg bw.
*P < 0.05, in comparison with C.

|
Protein expression and mRNA level of COX-2
After 14 days feeding, the protein expression and mRNA level of COX-2, which was primarily responsible for inflammation, were decreased by
L-methionine feeding. Compared to C, M62.5 (
P < 0.05), M75 (
P < 0.05) and M100 (
P < 0.05) produced dramatic decreases in the hepatic protein expression of COX-2 (
Fig. 3A), whereas no significant difference in the COX-2 protein level was observed between M50 and C (
P > 0.05,
Fig. 3A). The mRNA levels of
COX-2 were reduced markedly in groups M50 (
P < 0.05), M62.5 (
P < 0.05), M75 (
P < 0.05) and M100 (
P < 0.05) (
Fig. 3B), showing a good dose-dependent manner in
COX-2’s mRNA level. The results showed a significantly positive correlation between the hepatic HNE content and COX-2 expression (r = 0.8916,
P < 0.05), but a significantly negative correlation between
L-methionine intake and COX-2 expression (r = −0.8117,
P < 0.05). Overall, these findings suggested that
L-methionine suppresses HNE-induced inflammation.
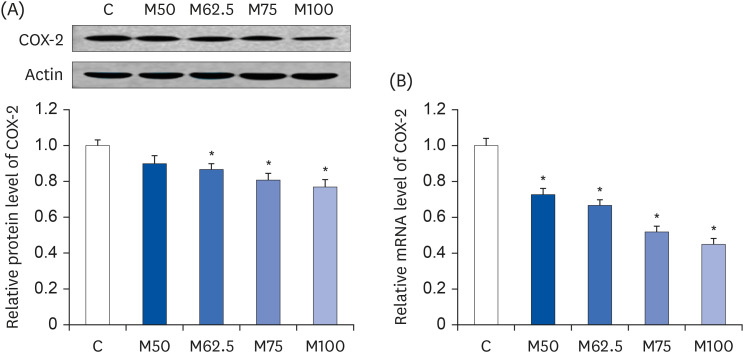 | Fig. 3
Hepatic protein expression and mRNA level of COX-2 after the oral administration of L-methionine for 14 days. (A) Hepatic protein expression of COX-2. (B) Hepatic mRNA level of COX-2. The values are expressed as the means ± SEM (n = 6).
COX-2, cyclooxygenase-2; C, control; M50, basal diet and administered L-methionine orally at 215.0 mg/kg bw; M62.5, basal diet and administered L-methionine orally at 268.8 mg/kg bw; M75, basal diet and administered L-methionine orally at 322.5 mg/kg bw; M100, basal diet and administered L-methionine orally at 430.0 mg/kg bw.
*P < 0.05, in comparison with C.

|
Protein expressions and mRNA levels of inflammatory mediators
After oral administration for 14 days,
L-methionine regulated the protein and mRNA levels of the inflammatory mediators. As summarized in
Fig. 4, the mRNA levels of
IL-1β (
P < 0.05,
Fig. 4A),
IL-6 (
P < 0.05,
Fig. 4B),
iNOS (
P < 0.05,
Fig. 4C) and
TNF-α (
P < 0.05,
Fig. 4D), which are major inflammatory mediators, were decreased markedly by
L-methionine. Compared to group C, the protein expression of IL-1β (
P < 0.05,
Fig. 4A), IL-6 (
P < 0.05,
Fig. 4B), iNOS (
P < 0.05,
Fig. 4C), and TNF-α (
P < 0.05,
Fig. 4D) were depressed significantly by M62.5, M75 and M100, whereas M50 did not induce significant increases in the protein expressions of IL-1β (
P > 0.05,
Fig. 4A), IL-6 (
P > 0.05,
Fig. 4B), iNOS (
P > 0.05,
Fig. 4C), and TNF-α (
P > 0.05,
Fig. 4D) compared to C.
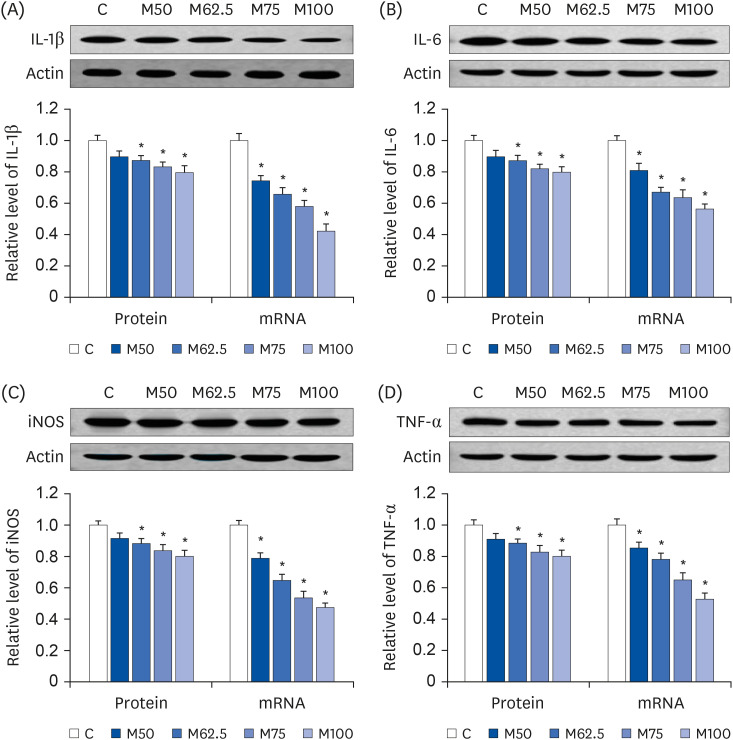 | Fig. 4
Hepatic protein expression and mRNA levels of inflammatory mediators after the oral administration of L-methionine for 14 days. (A) Hepatic protein expression and mRNA levels of IL-1β. (B) Hepatic protein expressions and mRNA levels of IL-6. (C) Hepatic protein expressions and mRNA levels of iNOS. (D) Hepatic protein expressions and mRNA levels of TNF-α. Values are the means ± SEM (n = 6).
IL-1β, interleukin-1β; IL-6, interleukin-6; iNOS, inducible nitric oxide synthase; TNF-α, tumor necrotic factor-alpha; C, control; M50, basal diet and administered L-methionine orally at 215.0 mg/kg bw; M62.5, basal diet and administered L-methionine orally at 268.8 mg/kg bw; M75, basal diet and administered L-methionine orally at 322.5 mg/kg bw; M100, basal diet and administered L-methionine orally at 430.0 mg/kg bw.
*P < 0.05, compared with C.

|
In this study, there were significantly positive correlations between the hepatic HNE contents and the inflammatory mediator expression of TNF-α (r = 0.8697, P < 0.05), of IL-1β (r = 0.8338, P < 0.05), of IL-6 (r = 0.8988, P < 0.05), and of iNOS (r = 0.8157, P < 0.05), confirming that HNE is an important inducer of inflammation. In contrast, the results showed significantly negative correlations between L-methionine intake and the expression of TNF-α (r = −0.8187, P < 0.05), IL-1β (r = −0.7646, P < 0.05), IL-6 (r = −0.8279, P < 0.05), and iNOS (r = −0.7566, P < 0.05), respectively. These results suggest that L-methionine could prevent HNE-derived inflammation, which is dependent on the L-methionine availability.
Protein expression and mRNA levels of NF-κB
After oral administration for 14 days, the pronounced decreases in the mRNA levels of
NF-κB1 (
P < 0.05,
Fig. 5A) and
RelA (
P < 0.05,
Fig. 5B), the major master regulators in the inflammatory process, were induced by
L-methionine. Compared to group C, the hepatic protein levels of NF-κB1 (
Fig. 5A) in groups M62.5, M75, and M100 were reduced markedly by 13.23% (
P < 0.05), 18.18% (
P < 0.05), and 20.63% (
P < 0.05), respectively after 14 days feedings, whereas M50 showed only a 10.76% decrease (
P > 0.05) in the protein expression of NF-κB1 with respect to C. As shown in
Fig. 5B, dramatic decreases in RelA protein expressions were found in M50 (
P < 0.05), M62.5 (
P < 0.05), M75 (
P < 0.05), and M100 (
P < 0.05), showing that the protein expressions of RelA were inhibited significantly by increasing the
L-methionine intake. The results showed significant negative correlations between the
L-methionine intake and expressions of NF-κB1 (r = −0.8027,
P < 0.05) and RelA (r = −0.8187,
P < 0.05).
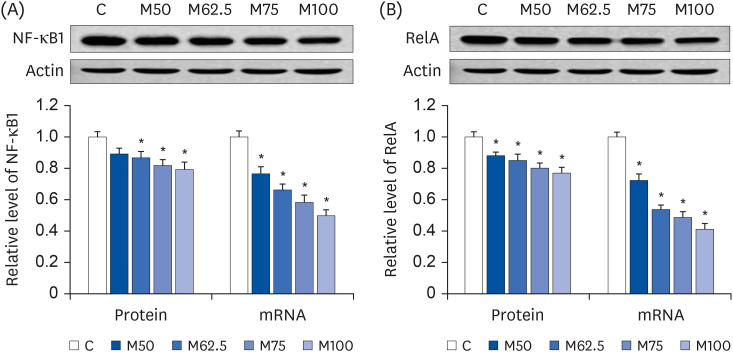 | Fig. 5
Hepatic protein expressions and mRNA levels of NF-κB1 and RelA after the oral administration of L-methionine for 14 days. (A) Hepatic protein expressions and mRNA levels of NF-κB1. (B) Hepatic protein expressions and mRNA levels of RelA. The values are expressed as the means ± SEM (n = 6).
NF-κB1, nuclear factor-κB1; RelA, reticuloendotheliosis viral oncogene homolog A; C, control; M50, basal diet and administered L-methionine orally at 215.0 mg/kg bw; M62.5, basal diet and administered L-methionine orally at 268.8 mg/kg bw; M75, basal diet and administered L-methionine orally at 322.5 mg/kg bw; M100, basal diet and administered L-methionine orally at 430.0 mg/kg bw.
*P < 0.05, compared with C.

|
Activation of NF-κB
This study examined the effects of
L-methionine on the NF-κB signaling pathway after 14 days of feeding. With the intake of
L-methionine, the protein expression (
Fig. 6A) and mRNA level (
Fig. 6B) of IκBα, which was an inhibitor of NF-κB, was increased gradually by
L-methionine administration in growing rats. Compared to group C, both protein expression (
Fig. 6A) and mRNA level (
Fig. 6B) of IκBα were increased markedly by M50 (
P < 0.05), M62.5 (
P < 0.05), M75 (
P < 0.05), and M100 (
P < 0.05), showing a significant positive correlation between the
L-methionine intake and IκBα expression (r = 0.8315,
P < 0.05).
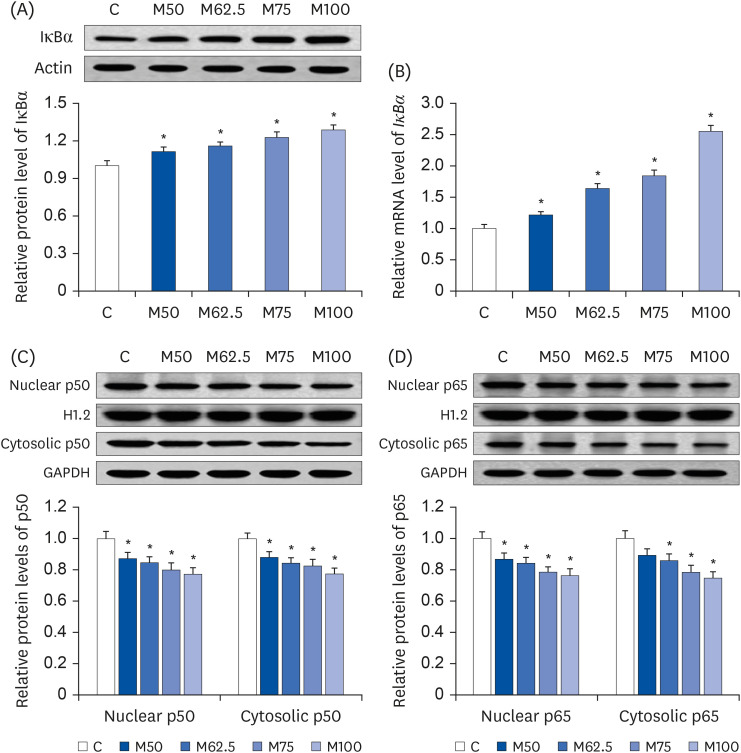 | Fig. 6
Effects of L-methionine on nuclear factor-κB activation after the oral administration for 14 days. (A) Hepatic protein expressions of IκBα. (B) Hepatic mRNA levels of IκBα. (C) Nuclear and cytosolic protein contents of p50. (D) Nuclear and cytosolic protein contents of p65. The values are expressed as the means ± SEM (n = 6).
IκBα, inhibitory κBα; C, control; M50, basal diet and administered L-methionine orally at 215.0 mg/kg bw; M62.5, basal diet and administered L-methionine orally at 268.8 mg/kg bw; M75, basal diet and administered L-methionine orally at 322.5 mg/kg bw; M100, basal diet and administered L-methionine orally at 430.0 mg/kg bw.
*P < 0.05, in comparison with C.

|
As a result, the dramatic decreases in the nuclear proportion of p50 (
Fig. 6C) and p65 (
Fig. 6D) were produced by M50 (
P < 0.05), M62.5 (
P < 0.05), M75 (
P < 0.05), and M100 (
P < 0.05), inducing the significant inhibition of nuclear translocation of p50 and p65 after
L-methionine feeding. In addition, the protein expressions of cytosolic p50 (
Fig. 6C) were reduced significantly by M50 (
P < 0.05), M62.5 (
P < 0.05), M75 (
P < 0.05), and M100 (
P < 0.05). Compared to C, the cytosolic p65 protein contents (
Fig. 6D) were increased significantly in groups M62.5 (
P < 0.05), M75 (
P < 0.05), and M100 (
P < 0.05), whereas group M50 did not produce a significant increase in the cytosolic p65 protein content (
P > 0.05) compared to group C.
Significant positive correlations were observed between the hepatic HNE content and the nuclear expression of p50 (r = 0.9069, P < 0.05) and p65 (r = 0.9198, P < 0.05). In contrast, significant negative correlations were observed between the L-methionine intake and nuclear contents of p50 (r = −0.9178, P < 0.05) and p65 (r = −0.9285, P < 0.05). Overall, these results suggested that L-methionine can depress HNE-derived NF-κB activation.
Protein expression and mRNA levels of PI3K and AKT
The expression of PI3K and AKT, which could regulate the NF-kB signaling pathway, was investigated to determine if
L-methionine could depress the activation of NF-κB. Compared to group C, the mRNA levels of
PI3K (
Fig. 7A) and
AKT (
Fig. 7B) were markedly reduced in groups M50 (
P < 0.05), M62.5 (
P < 0.05), M75 (
P < 0.05), and M100 (
P < 0.05), respectively. The protein expression of PI3K and AKT was also attenuated by
L-methionine intake. The dramatic depressions on PI3K protein expression (
Fig. 7A) were found in M50 (
P < 0.05), M62.5 (
P < 0.05), M75 (
P < 0.05), and M100 (
P < 0.05). As shown in
Fig. 7B, the protein expression of AKT was reduced significantly by M62.5 (
P < 0.05), M75 (
P < 0.05), and M100 (
P < 0.05), whereas no significant difference was found between M50 and C (
P > 0.05). Overall,
L-methionine feeding can decrease the gene and protein expression of PI3K and AKT, which are dependent on
L-methionine availability.
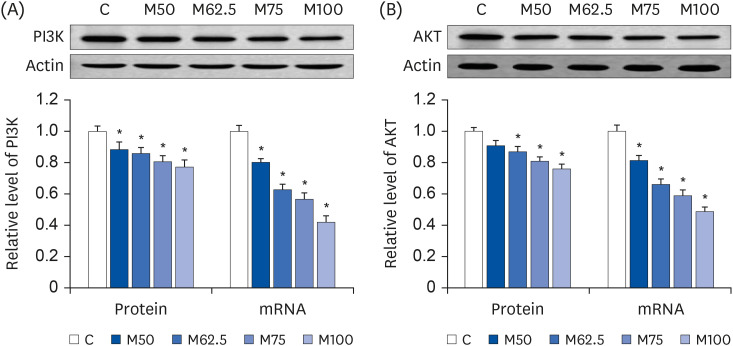 | Fig. 7
Hepatic protein expressions and mRNA levels of PI3K and AKT after the oral administration of L-methionine for 14 days. (A) Hepatic protein expressions and mRNA levels of PI3K. (B) Hepatic protein expressions and mRNA levels of AKT. The values are expressed as the means ± SEM (n = 6).
PI3K, phosphoinositide 3 kinase; AKT, protein kinase B; C, control; M50, basal diet and administered L-methionine orally at 215.0 mg/kg bw; M62.5, basal diet and administered L-methionine orally at 268.8 mg/kg bw; M75, basal diet and administered L-methionine orally at 322.5 mg/kg bw; M100, basal diet and administered L-methionine orally at 430.0 mg/kg bw.
*P < 0.05, compared with C.

|
In this study, negative correlations were observed between L-methionine intake and the expression of PI3K/AKT (PI3K, r = −0.8434, P < 0.05; AKT, r = −0.7983, P < 0.05). Furthermore, significant positive correlations were noted between the nuclear contents of NF-κB and expression of PI3K (p50, r = 0.9410, P < 0.05; p65, r = 0.9503, P < 0.05) and AKT (p50, r = 0.7968, P < 0.05; p65, r = 0.8079, P < 0.05).
Protein expressions and mRNA levels of IL-10 and GST
After 14 days of feeding,
L-methionine regulated the protein expression and mRNA level of IL-10, which was an anti-inflammatory mediator. As shown in
Fig. 8A, compared to group C, the protein expressions of IL-10 were augmented significantly in groups M50 (
P < 0.05), M62.5 (
P < 0.05), M75 (
P < 0.05), and M100 (
P < 0.05). The mRNA levels of
IL-10 were also increased markedly enhanced in groups M50 (
P < 0.05), M62.5 (
P < 0.05), M75 (
P < 0.05), and M100 (
P < 0.05), with increases ranging from 29.23% to 126.56% (
Fig. 8B). The results showed a significant positive correlation between
L-methionine intake and the expression of IL-10 (r = 0.9225,
P < 0.05).
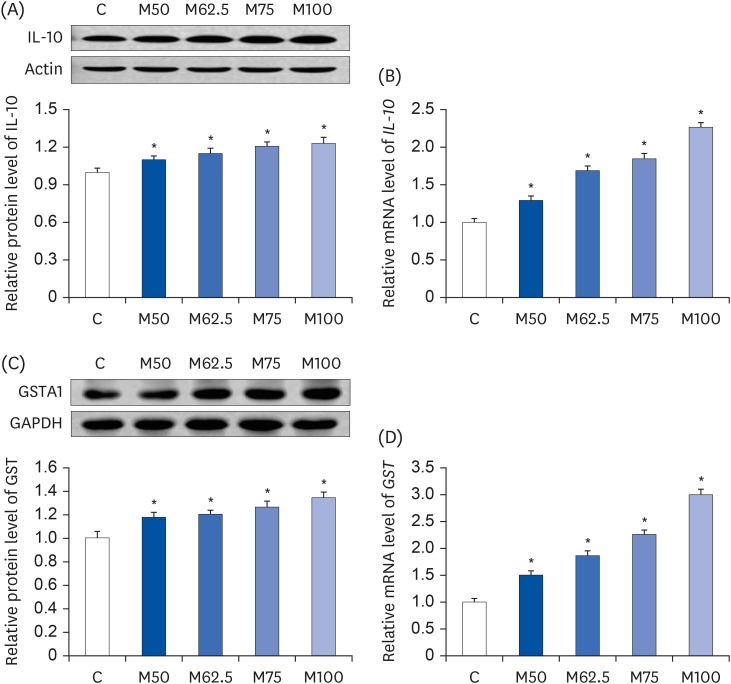 | Fig. 8
Hepatic protein expression and mRNA levels of IL-10 and GST in growing rats. (A) Hepatic protein expressions of IL-10. (B) Hepatic mRNA levels of IL-10. (C) Hepatic protein expressions of GST. (D) Hepatic mRNA levels of GST. The values are expressed as the means ± SEM (n = 6).
IL-10, interleukin-10; GST, glutathione S-transferase α1; C, control; M50, basal diet and administered L-methionine orally at 215.0 mg/kg bw; M62.5, basal diet and administered L-methionine orally at 268.8 mg/kg bw; M75, basal diet and administered L-methionine orally at 322.5 mg/kg bw; M100, basal diet and administered L-methionine orally at 430.0 mg/kg bw.
*P < 0.05, compared with C.

|
Similarly, the protein expressions (
Fig. 8C) and mRNA levels (
Fig. 8D) of GST, which could exert a HNE-scavenging action, were elevated dramatically by M50 (
P < 0.05), M62.5 (
P < 0.05), M75 (
P < 0.05), and M100 (
P < 0.05). Compared to group C, the protein expression of GST was stimulated by
L-methionine in a dose-dependent manner (
Fig. 8C). In addition, the mRNA levels of
GST (
Fig. 8D) were stimulated by
L-methionine intake, with increases ranging from 50.13% (M50,
P < 0.05) to 199.76% (M100,
P < 0.05) in growing rats.
Go to :

DISCUSSION
The regulatory effect of
L-methionine on inflammation was investigated in this study. After oral administration for 14 days,
L-methionine had anti-inflammatory effects in growing rats. As major inflammatory biomarkers, the expression of COX-2, TNF-α, IL-1β, IL-6, and iNOS revealed the inflammatory process [
2425]. Thus, the depression of COX-2, TNF-α, IL-1β, IL-6, and iNOS expressions was suggested to be a useful target for preventing inflammation. Hence, in this study, the anti-inflammatory action of
L-methionine was reflected primarily by the down-regulated expression of these inflammatory mediators in growing rats. Moreover,
L-methionine feeding could enhance the expression of IL-10, which is an important anti-inflammatory mediator [
24]. Thus, the present study showed that
L-methionine feeding could depress the inflammation in growing rats, which is dependent on the availability of
L-methionine.
Here, the question might arise as to why
L-methionine could exert an anti-inflammatory function after 14 days of feeding. To answer this question, the view that oxidative stress can initiate an inflammatory process should be considered first. HNE, which is a major end product of lipid peroxidation, can induce an inflammatory process by stimulating COX-2 expression [
5]. In this study, hepatic HNE contents were significantly reduced by
L-methionine feeding. More significantly, the decreased expression of the inflammatory mediators (COX-2, IL-1β, IL-6, TNF-α, and iNOS) was attributed to the decreased HNE accumulation, confirming that HNE is an important inducer of inflammation. Thus,
L-methionine can inhibit HNE accumulation and prevent HNE-derived inflammation.
The view that scavenging reactive oxygen species (ROS) is a key step to inhibiting HNE-induced inflammation has merit because HNE is produced by oxidative stress [
56].
L-methionine may have antioxidant capacity [
2627]. In particular, in this series of studies,
L-methionine could scavenge ROS [
17182228], suggesting that
L-methionine might decrease the formation of HNE. In this study, with oral administration, the inhibitory effect of
L-methionine on hepatic HNE accumulation was observed in growing rats fed M50, M62.5, M75, or M100. Accordingly, the depression of HNE-induced inflammation resulted from attenuating the ROS-induced oxidative damage to lipids in growing rats fed with
L-methionine.
This study examined the role of
L-methionine in HNE-scavenging action to explain why
L-methionine could exert an anti-inflammatory function after 14 days of feeding. With its strong hydrophobicity, HNE can react with DNA, proteins, and other molecules that contain nucleophilic thiol (-SH) or amino (-NH
2) groups. Principally, HNE can bind to three different side-chain amino acids, i.e., cysteine (Cys), histidine (His), and lysine (Lys), to form Michael adducts via Michael addition, in which cysteine exhibits the strongest reactivity [
2930]. Thus,
L-methionine feeding could stimulate the transsulfuration pathway to increase the supply of cysteine [
17], which can exert HNE-scavenging activity. Moreover, for a low-molecular compound, hydrogen sulfide (H
2S) can also react with HNE to exert protective activity against HNE-induced toxicity. Increasing evidence suggests that the HNE formation can be inhibited markedly by the increased synthesis of endogenous H
2S, which is produced by the transsulfuration pathway [
2630]. Therefore, a satisfactory explanation can be further drawn from the authors’ series of studies, in which
L-methionine feeding effectively stimulated the expression of cystathionine β-synthase (CBS) and cystathionine γ-lyse (CTH) to increase the supply of cysteine and promote H
2S synthesis [
171822]. Accordingly, the HNE-scavenging activity could be enhanced by increasing the intake of
L-methionine, leading to an anti-inflammatory action in growing rats after 14 days of feeding.
The inflammatory process can be inhibited by dietary antioxidants [
24]. Hence, the antioxidant effects of
L-methionine must also be noted to explain the findings of this study. Previous studies reported the molecular antioxidant mechanism exerted by
L-methionine [
171822]. Wang
et al. [
17] stated that
L-methionine could activate Nrf2, a key transcription factor for antioxidant mechanism, and up-regulated ARE-dependent gene expression to scavenge ROS. The results showed that
L-methionine could induce the endogenous antioxidant response by increasing GSH synthesis to depress ROS-derived oxidative stress. As an endogenous antioxidant, GSH can bind HNE rapidly, showing a HNE-detoxifying effect of GSH [
429303132]. Furthermore, as a major determinant of the intracellular concentration of HNE, GST also plays a role in detoxification. GST regulates the HNE metabolism via its conjugation to GSH, resulting in the depression of HNE formation during lipid peroxidation [
303334]. Overall,
L-methionine could decrease the HNE level, depending on enhanced GSH synthesis and stimulated GST expression exerted by
L-methionine. In addition, upon the activation of Nrf2,
L-methionine could stimulate the gene and protein expression of heme oxygenase 1 (HO-1) [
17182223], which was another anti-inflammatory mediator [
1324]. Thus,
L-methionine can depress the HNE-induced inflammation, possibly due to the stronger antioxidant capacity of
L-methionine.
The regulatory effect of
L-methionine on the NF-κB pathway was investigated to explain how
L-methionine can depress the inflammatory process after oral administration for 14 days. The major inflammatory mediators, e.g., COX-2, TNF-α, IL-1β, IL-6, and iNOS, can be up-regulated with NF-κB activation [
925]. Thus, NF-κB is a master regulator for the inflammatory process and plays a crucial role in initiating inflammation [
9]. Upon the activation of NF-κB, the major complexes of NF-κB, e.g., p50 and p65, can translocate to the nucleus, initiating an inflammatory process [
935]. In this study,
L-methionine could inhibit the activation of NF-κB by stimulating IκBα expression, which was an inhibitor of NF-κB [
9]. More importantly, the significant finding was that
L-methionine could inhibit the translocation of p50 and p65 to the nucleus. Consequently, the inflammatory response was inhibited by
L-methionine feeding by depressing NF-κB activation.
The activation of NF-κB is closely linked to oxidative damage to lipids [
2435]. As a critical marker of lipid peroxidation, HNE can regulate NF-κB activation [
78]. The findings observed in this study are also consistent with this view. Therefore, the present study confirmed that
L-methionine could depress NF-κB activation, which might be because of the decreased HNE accumulation by
L-methionine, confirming that
L-methionine could depress HNE-induced inflammation in growing rats.
NF-κB activation was also regulated by PI3K/AKT. As one of the targets of the PI3K/AKT pathway, NF-κB signaling can be activated via the up-regulation of PI3K/AKT [
363738]. Wang
et al. reported that the down-regulation of PI3K/AKT might be a switch to the depression of NF-κB activation involving the anti-inflammatory action exerted by rice protein [
24]. Consistent with this view, this study found that the decreased nuclear contents of NF-κB were closely linked to the decreased expression of PI3K/AKT in growing rats fed
L-methionine. Nevertheless, the present study clearly showed that the anti-inflammatory action of
L-methionine can be explained partly by the depression of NF-κB activation via down-regulating PI3K/AKT.
In this study, L-methionine affected the anti-inflammatory action in a dose-dependent manner. With the lowest level of L-methionine, the hepatic HNE level was higher in the M50 group than in the M62.5, M75, or M100 groups. As a result, the protein expression and mRNA levels of the inflammatory mediators (COX-2, IL-1β, IL-6, TNF-α, and iNOS) were higher in the M50 group than in the M62.5, M75, or M100 groups. In contrast, the protein expression and mRNA level of the anti-inflammatory mediator (IL-10) were lower in the M50 group than in the M62.5, M75, or M100 groups. In this study, of interest was the finding that the M50 group showed significantly lower mRNA levels (P < 0.05) of the inflammatory mediators (COX-2, IL-1β, IL-6, TNF-α, iNOS, and AKT) compared to the control group. On the other hand, compared to the marked magnitude of the change in the mRNA levels (P < 0.05, the changes in the protein levels of these inflammatory mediators (COX-2, IL-1β, IL-6, TNF-α, iNOS, and AKT) were not large in the M50 group in compared to the control group (P > 0.05). This absence of a protein-mRNA correlation for investigated levels of genes and proteins of the inflammatory mediators in this study might be due to the relationship between the protein and mRNA, which is not strictly linear. Moreover, under the present experimental condition, M100 exhibited the strongest anti-inflammatory activity, whereas the weakest anti-inflammatory effect was induced by M50. The influence of L-methionine on the antioxidative capacity should be considered when explaining this phenomenon. These findings highlighted the critical role of L-methionine in the ROS-scavenging activity and activating the endogenous antioxidant system via the Nrf2-ARE pathway to inhibit lipid oxidation, which is dependent on the availability of L-methionine. A link between the higher composition of L-methionine and stronger ROS-derived HNE scavenging activity is conceivable. This is supported by the lower protein expression and mRNA level of GST, which could exert HNE-scavenging activity, in the M50 group than in the M62.5, M75, or M100 groups. The present study confirmed that higher levels of L-methionine might possess a stronger anti-inflammatory function.
In summary,
L-methionine can inhibit hepatic HNE accumulation and depress HNE-derived inflammation in growing rats, which is dependent on the availability of
L-methionine. With the oral administration of
L-methionine, the hepatic HNE-scavenging activity was attributed to the stimulation of GST by
L-methionine. With the decreased HNE level, the anti-inflammatory action is reflected by the suppressed expression of the inflammatory biomarkers (COX-2, TNF-α, IL-1β, IL-6, and iNOS) and the up-regulation of the anti-inflammatory mediator (IL-10). Of significance is the finding that the molecular mechanism underlying the anti-inflammatory capacity of
L-methionine is to inhibit NF-κB activation by down-regulating PI3K/AKT (
Fig. 9). Nevertheless, more detailed investigations will be needed to explore the precise mechanisms exerted by
L-methionine to prevent inflammation.
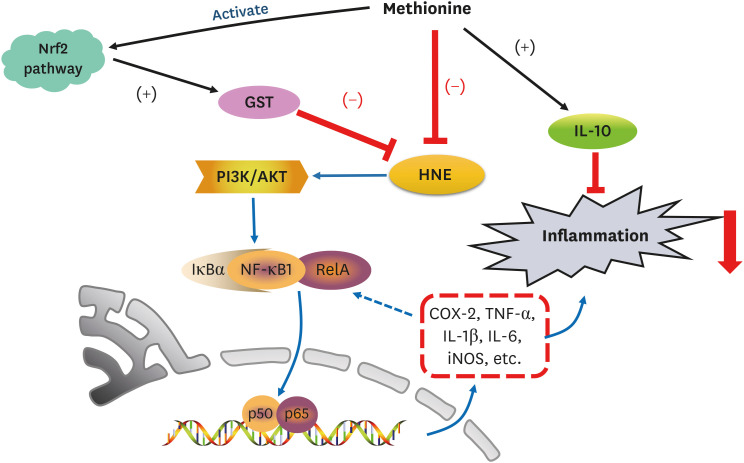 | Fig. 9
L-Methionine inhibits HNE accumulation and depresses inflammation by inhibiting NF-κB activation and up-regulating GST.
Nrf2, nuclear factor erythroid 2-related factor 2; GST, glutathione S-transferase; PI3K, phosphoinositide 3 kinase; AKT, protein kinase B; HNE, 4-hydroxy-2-nonenal; IκBα, inhibitory κBα; NF-κB, nuclear factor-κB; COX-2, cyclooxygenase-2; TNF-α, tumor necrotic factor alpha; IL, interleukin; iNOS, inducible nitric oxide synthase.

|
Go to :
