INTRODUCTION

MATERIALS AND METHODS
Preparation of ADLE
Cell culture and treatment
Animal experiments
Islet isolation
Histology of pancreas tissues
Assay for apoptotic cell death
Cellular lipids measurement
Measurement of insulin secretion
Measurement of the nitrite content
Measurement of intracellular reactive oxygen stress
Measurement of cellular ATP
Western blot analysis
Quantitative real-time polymerase chain reaction (qRT-PCR)
Table 1
Primer sequences for real-time PCR
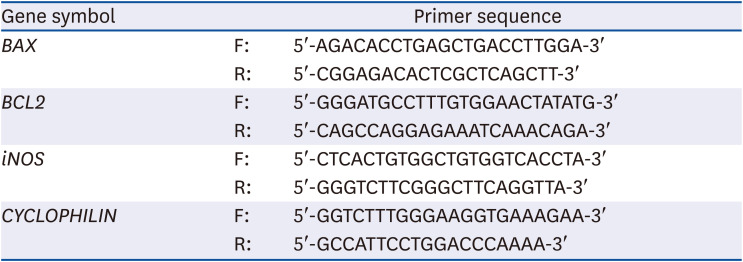
Statistical analysis

RESULTS
ADLE reduces oxidative stress and apoptosis in the islets from HFD-induced diabetic mice
![]() | Fig. 1ADLE reduces oxidative stress and apoptosis in the islets from high fat diet-induced diabetic C57BL/6J mice. (A) Representative photomicrographs of immune-staining for insulin (brown color) in pancreatic islets. (B) Measurement of the density in the islet area (pixel). (C) Representative photomicrographs of mice pancreatic sections stained with the 4-HNE antibody. (D) Representative photomicrographs of TUNEL-positive cells in pancreatic sections. (E) Histogram representing the quantitative analysis of TUNEL-positive β cells per islet in each experimental group. (F) Plasma serum level at 0 and 30 min after glucose injection (2 g/kg) collected for insulin detection with ELISA (magnification: ×200). Data are presented as the mean ± SD (n = 5–8). The arrows indicated the 4-HNE and TUNEL stained cells. The significance was evaluated using ANOVA with the LSD comparisons test.ADLE, Allomyrina dichotoma larva extract; NFD, normal fat diet group; HFD, high-fat diet group; HFD+ADLE, high-fat diet plus 100 mg/kg/day ADLE; 4-HNE, 4-hydroxynonenal; TUNEL, terminal deoxynucleotidyl transferase dUTP nick end labeling; ANOVA, analysis of variance; LSD, Least Significant Difference; ns, no significance.
*P < 0.05 and **P < 0.01 versus NFD; #P < 0.05 versus HFD.
|
ADLE attenuates cytotoxicity and lipid accumulation in palmitate-stimulated INS-1 cells
![]() | Fig. 2ADLE attenuates cytotoxicity and lipid accumulation in palmitate-stimulated INS-1 cells. (A) Effects of ADLE on the proliferation of INS-1 cells. The cells were incubated in media containing various concentrations of ADLE (0.1, 0.25, 0.5, 1.0, and 2.0 mg/mL) for 24 h. The cell viability was measured using an MTT assay. (B) INS-1 cells were treated with 0.4 mM PAL with or without 0.1, 0.25, and 0.5 mg/mL of ADLE. (C) Rat pancreatic islets were treated with 0.4 mM PAL with or without 0.5 mg/mL of ADLE. The cell viability at 24 h was determined using an MTT assay. (D) The intracellular lipid levels were measured using a triglyceride assay kit. The values are presented as the mean ± SD (n = 3–5). The significance was evaluated using ANOVA with the LSD comparisons test.ADLE, Allomyrina dichotoma larva extract; CON, untreated control; PAL, 0.4 mM palmitate only; CON+ADLE, untreated control plus 0.5 mg/mL ADLE; PAL+ADLE, 0.4 mM palmitate plus 0.5 mg/mL ADLE; MTT, methyl thiazolyl diphenyl tetrazolium; TG, triglyceride; ANOVA, analysis of variance; LSD, Least Significant Difference; ns, no significance.
*P < 0.05 and ***P < 0.001 versus CON; ##P < 0.01 and ###P < 0.001 versus PAL.
|
ADLE treatment partially improves insulin secretion via AMPK activation in INS-1 cells
![]() | Fig. 3ADLE treatment partially improves insulin secretion via AMPK activation in INS-1 cells. (A) Insulin release was measured after 2 h of incubation in either 3.3 mmol/L glucose or 25 mmol/L glucose, as described in materials and methods. (B) The expression levels of total-AMPK and phospho-AMPK were measured by western blot analysis. β-actin was used as the loading control. The bands were quantified using Quantity 1 software. The data are presented as the mean ± SD (n = 3–5). The significance was evaluated using an ANOVA with the LSD comparisons test.ADLE, Allomyrina dichotoma larva extract; CON, untreated control; PAL, 0.4 mM palmitate only; CON+ADLE, untreated control plus 0.5 mg/mL ADLE; PAL+ADLE, 0.4 mM palmitate plus 0.5 mg/mL ADLE; AMPK, AMP-activated protein kinase; ANOVA, analysis of variance; LSD, Least Significant Difference.
*P < 0.05, **P < 0.01, and ***P < 0.001 versus CON, #P < 0.05 and ###P < 0.001 versus PAL.
|
ADLE treatment reduces palmitate-induced apoptosis in INS-1 cells
![]() | Fig. 4ADLE treatment reduces the expression level of the apoptosis-associated proteins and DNA fragmentation in palmitate-treated INS-1 cells. (A) INS-1 cells were treated with 0.5 mg/mL ADLE and 0.4 mM PAL. The cells were evaluated 24 h after treatment. The whole protein lysates were prepared using an extraction buffer. The levels of caspase-3 (A) and PARP (B) were determined by Western blot analysis with anti-caspase-3 and anti-PARP antibodies, respectively. (C) Fragmented DNA was then measured using a Cell Death Detection ELISA kit. The data are presented as the mean ± SD (n = 3–5). β-actin was used as the loading control. The bands were quantified using Quantity 1 software. Significance was evaluated using an ANOVA with an LSD comparisons test.ADLE, Allomyrina dichotoma larva extract; CON, untreated control; PAL, 0.4 mM palmitate only; CON+ADLE, untreated control plus 0.5 mg/mL ADLE; PAL+ADLE, 0.4 mM palmitate plus 0.5 mg/mL ADLE; PARP, poly (ADP-ribose) polymerase; ELISA, enzyme-linked immunosorbent assay; ANOVA, analysis of variance; LSD, Least Significant Difference.
*P < 0.05, **P < 0.01, and ***P < 0.001 versus CON, #P < 0.05 and ##P < 0.01 versus PAL.
|
![]() | Fig. 5ADLE treatment reduces the Bax/Bcl2 ratio in palmitate-treated INS-1 cells. (A) INS-1 cells were treated with 0.4 mM PAL in the absence or presence of 0.5 mg/mL ADLE. The cells were harvested after 24 h of treatment. The mRNA levels of BCL2 and BAX proteins were analyzed by qRT-PCR. The mRNA levels were normalized with those of cyclophilin. (B) The cells were treated as described in (A) and harvested after a 24 h treatment. The levels of Bcl2 and Bax expression were measured by western blot analysis. β-actin was used as the loading control. The bands were quantified using Quantity 1 software. The values represent the mean ± SD (n = 3–5). The significance was evaluated using an ANOVA with the LSD comparisons test.ADLE, Allomyrina dichotoma larva extract; BCL2, B cell lymphoma 2; BAX, Bcl-2-associated X; CON, untreated control; PAL, 0.4 mM palmitate only; qRT-PCR, quantitative real-time polymerase chain reaction; CON+ADLE, untreated control plus 0.5 mg/mL ADLE; PAL+ADLE, 0.4 mM palmitate plus 0.5 mg/mL ADLE; ANOVA, analysis of variance; LSD, Least Significant Difference.
*P < 0.05, **P < 0.01, and ***P < 0.001 versus CON. #P < 0.05, ##P < 0.01, and ###P < 0.001 versus PAL.
|
ADLE treatment partially prevents ROS generation and restores the ATP levels reduced by palmitate in INS-1 cells
![]() | Fig. 6ADLE treatment partially prevents ROS generation and restores the ATP levels reduced by palmitate in INS-1 cells. INS-1 cells were treated with 0.4 mM PAL in the absence or presence of 0.5 mg/mL ADLE. The cells were harvested, and (A) ROS and (B) intracellular ATP levels were examined. Data represent the mean ± SD (n = 3–5). Significance was evaluated using an ANOVA with the LSD comparisons test.ADLE, Allomyrina dichotoma larva extract; CON, untreated control; PAL, 0.4 mM palmitate only; CON+ADLE, untreated control plus 0.5 mg/mL ADLE; PAL+ADLE, 0.4 mM palmitate plus 0.5 mg/mL ADLE; ROS, reactive oxygen species; ATP, adenosine triphosphate; ANOVA, analysis of variance; LSD, Least Significant Difference.
|
ADLE treatment reduces palmitate-induced iNOS expression and nitrite in INS-1 cells
![]() | Fig. 7ADLE treatment reduces palmitate-induced iNOS expression and nitrite in INS-1 cells. INS-1 cells were treated with 0.4 mM PAL in the absence or presence of 0.5 mg/mL ADLE. The cells were harvested after 16 h of treatment. (A) The nitrite content was measured in the cell-free culture supernatants. (B) The mRNA levels of iNOS were analyzed by quantitative RT-PCR. The mRNA levels were normalized to those of cyclophilin. (C, D) The iNOS protein level was determined by western blotting. The Western blotting data are representative of three independent experiments. The bands were quantified using Quantity 1 software. The significance was evaluated using an ANOVA with the LSD comparisons test. The data represent mean ± SD (n = 3–5).ADLE, Allomyrina dichotoma larva extract; CON, untreated control; PAL, 0.4 mM palmitate only; CON+ADLE, untreated control plus 0.5 mg/mL ADLE; PAL+ADLE, 0.4 mM palmitate plus 0.5 mg/mL ADLE; iNOS, inducible nitric oxide synthase; ANOVA, analysis of variance; LSD, Least Significant Difference.
|

DISCUSSION
