Abstract
BACKGROUND/OBJECTIVES
MATERIALS/METHODS
RESULTS
Figures and Tables
![]() | Fig. 1The mRNA levels of genes related to intestinal fatty acid uptake and channeling.Data are presented as mean ± SEM, n = 6 for each group. Two-way ANOVA was used to determine the significant effects of fat amount and oil type and was followed by a LSD post-hoc test. Different letters indicate significant difference at P < 0.05. *A significant overall effect of fat amount was observed in a two-way ANOVA, but no significant differences were observed among individual group comparisons in the post hoc test.
|
![]() | Fig. 2The mRNA levels of genes related to intestinal chylomicron assembly and secretion.Data are presented as mean ± SEM, n = 6 for each group. Two-way ANOVA was used to determine the significant effects of fat amount and oil type and was followed by a LSD post-hoc test. Different letters indicate significant difference at P < 0.05.
|
![]() | Fig. 3The mRNA levels of genes related to hepatic lipid uptake and channeling.Data are presented as mean ± SEM, n = 6 for each group. Two-way ANOVA was used to determine the significant effects of fat amount and oil type and was followed by a LSD post-hoc test. Different letters indicate significant difference at P < 0.05. *A significant overall effect of oil type was observed in a two-way ANOVA, but no significant differences among individual group comparisons were observed in the post hoc test.
|
![]() | Fig. 4The mRNA levels of genes related to hepatic TAG lipolysis and fatty acid oxidation.Data are presented as mean ± SEM, n = 6 for each group. Two-way ANOVA was used to determine the significant effects of fat amount and oil type and was followed by a LSD post-hoc test. Different letters indicate significant difference at P < 0.05.
|
![]() | Fig. 5The mRNA levels of genes related to hepatic TAG synthesis and VLDL assembly.Data are presented as mean ± SEM, n = 6 for each group. Two-way ANOVA was used to determine the significant effects of fat amount and oil type and was followed by a LSD post-hoc test. Different letters indicate significant difference at P < 0.05. *A significant overall effect of oil type was observed in a two-way ANOVA, but no significant differences were observed among individual group comparisons in the post hoc test.
|
Table 1
Composition of the experiment diets1)
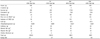
1)Resource: Dyets, Inc, Bethlehem, PA, USA.
2)PNO was a gift from the Dubio Co., Ltd. (Hwaseong-City, GyeongGi-do, Korea)
3)Thirty-five grams of mineral mix (Dyets, #210099) provides 5.1 g calcium, 4 g phosphorus, 3.6 g potassium, 1 g sodium, 1.6 g chloride, 0.5 g magnesium, 0.3 g sulfur, 59 mg manganese, 46 mg iron, 25 mg zinc, 5 mg copper, 0.2 mg selenium, 0.2 mg iodine and 4.2 g sucrose.
4)Ten grams of vitamin mix (Dyets, #300050) provides 4,000 IU vitamin A, 1,000 IU vitamin D3, 50 IU vitamin E, 30 mg niacin, 16 mg pantothenic acid, 7 mg vitamin B6, 6 mg vitamin B1, 6 mg vitamin B2, 2 mg folic acid, 0.8 mg menadione, 0.2 mg biotin, 10 µg vitamin B12 and 9.8 g sucrose.
Table 4
The primer sequences for hepatic genes used in real-time PCR
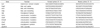
Lrp1: low density lipoprotein receptor-related protein 1; Fatp5: Fatty acid transporter 5; Acsl1: acyl-CoA synthetase long-chain family member 1; Acbp: Acyl-CoA-binding protein; Atgl: Adipose triglyceride lipase; Cpt1a: carnitine palmitoyltransferase 1a; Acadl: Long Chain Acyl-CoA Dehydrogenase; Ehhadh: enoyl-Coenzyme A, hydratase/3-hydroxyacyl Coenzyme A dehydrogenase; Acaa1: acetyl-Coenzyme A acyltransferase 1a; Dgat2: diacylglycerol O-acyltransferase 2; ApoB100: apolipoprotein B-100.
Table 5
Body weight, weight gain, white adipose tissue weight and liver weight1)

1)Two-way ANOVA was used to determine the significant effects of fat amount and oil type and was followed by a LSD post-hoc test. Different letters indicate significant difference at P < 0.05. Data are presented as mean ± SEM.
2)Liver-to-body weight ratio (%) = 100% *liver weight (mg) / body weight (g)
References
































