Abstract
Objective
Methods
Results
Conclusion
Notes
References









































Fig. 1.
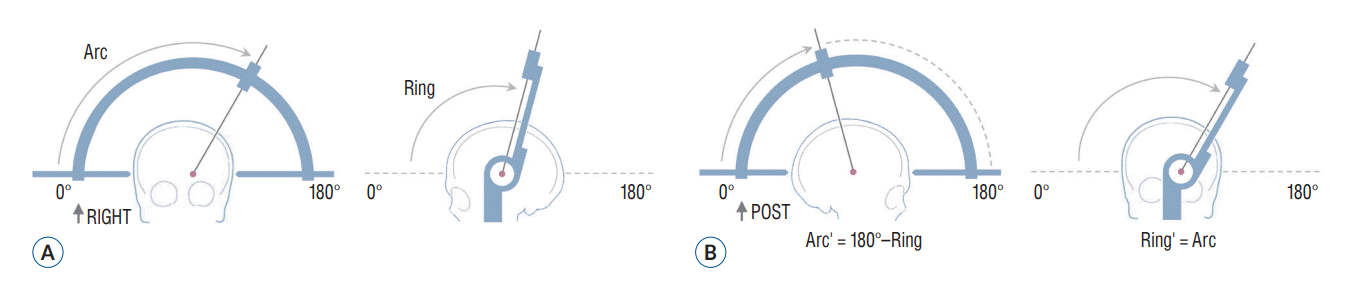
Fig. 2.
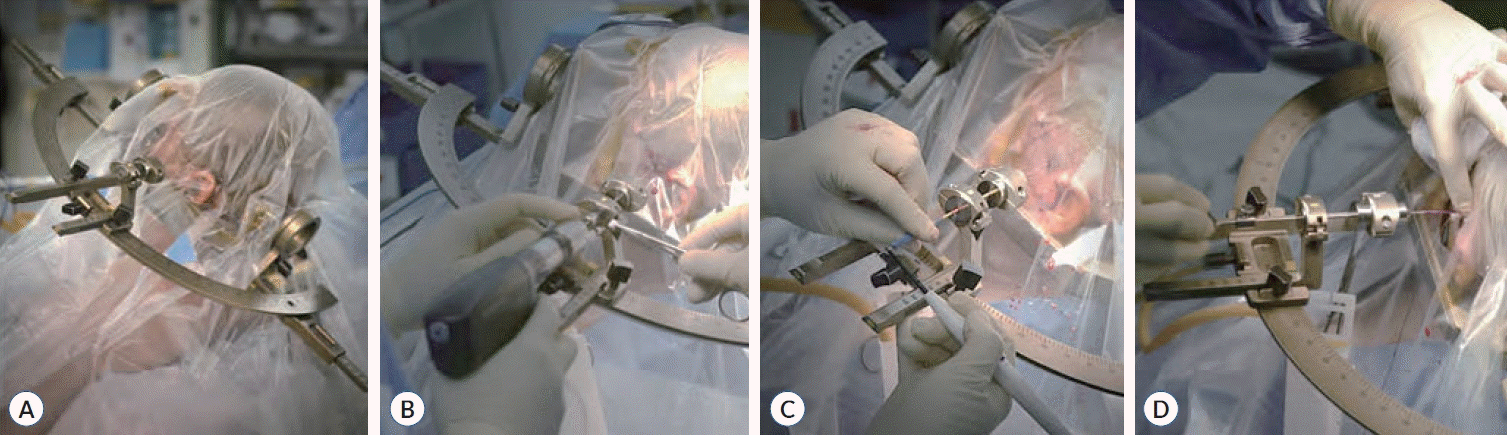
Fig. 3.
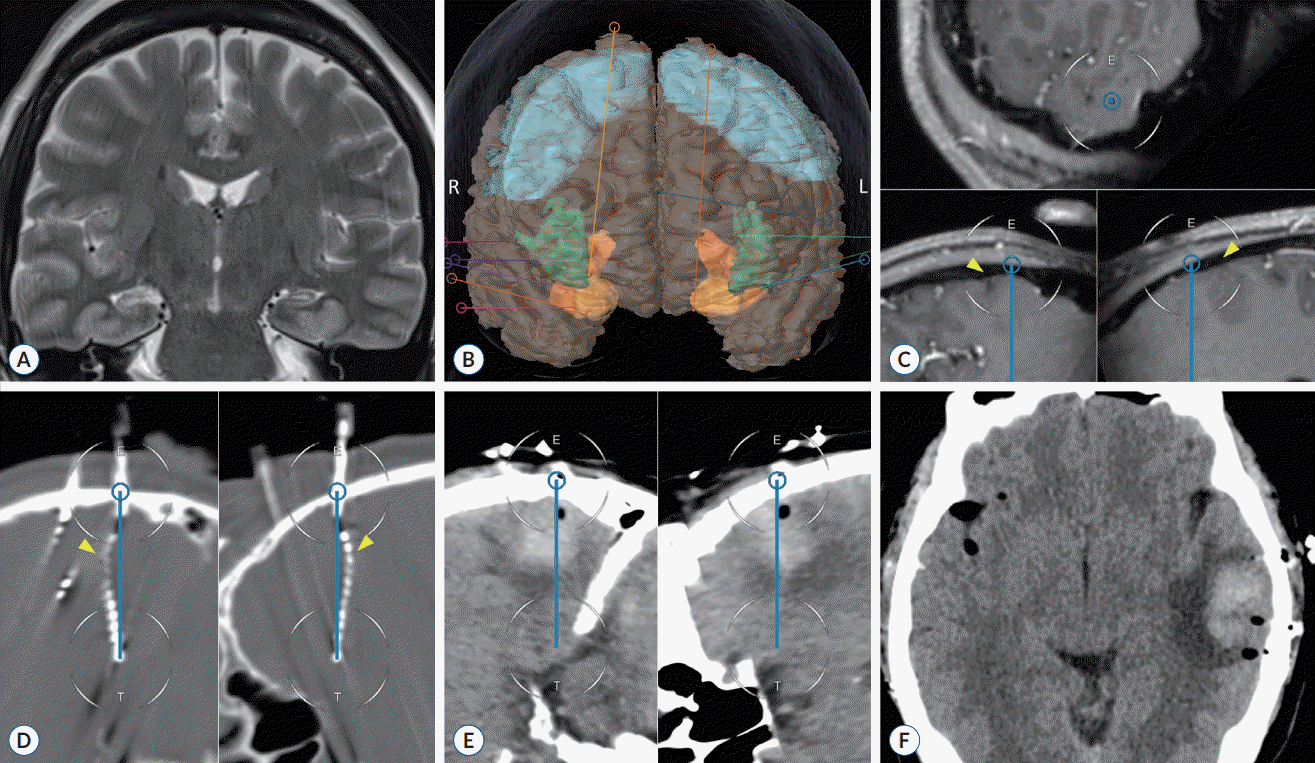
Fig. 4.
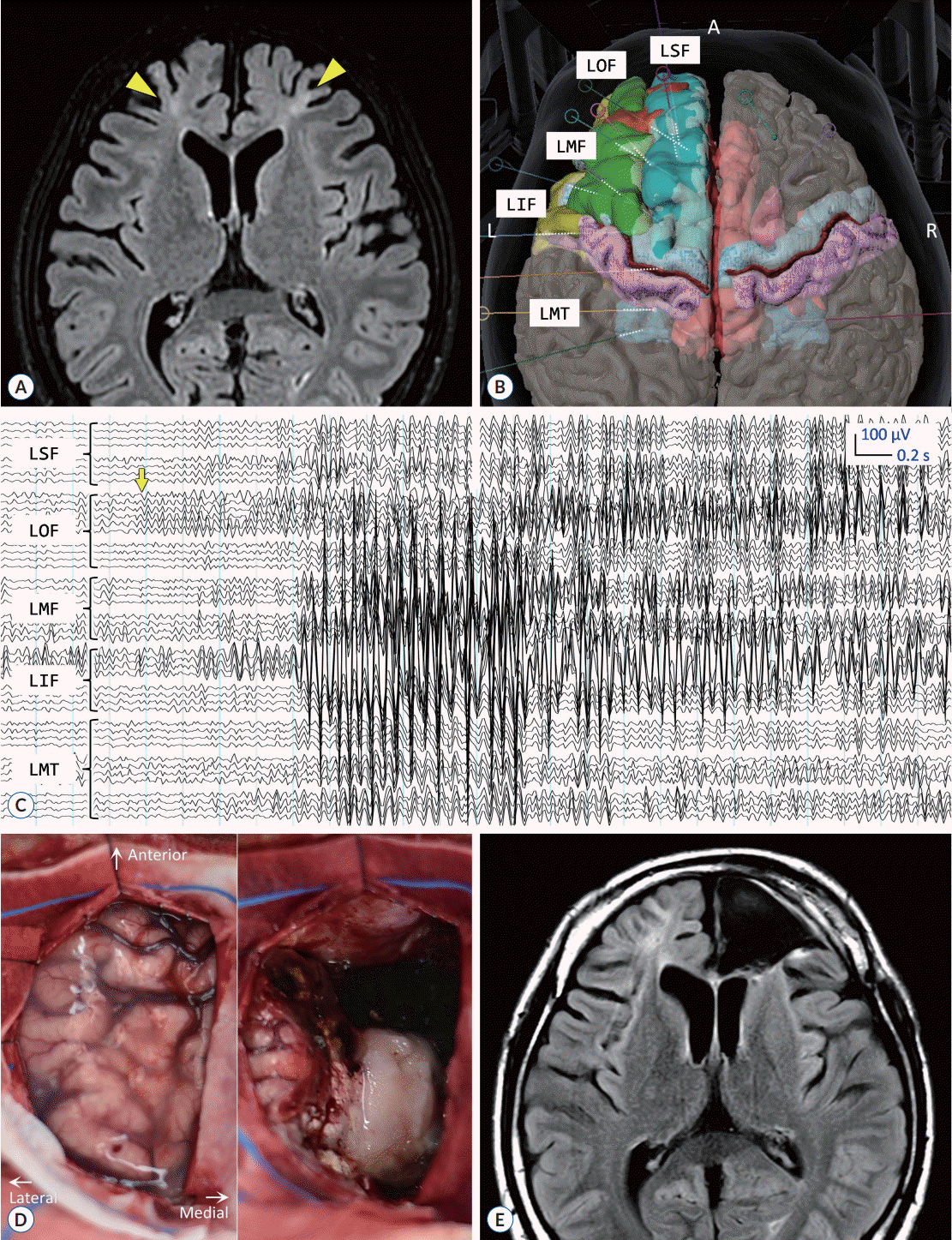
Table 1.
Case | Sex/age (years) | Onset age (duration) (years) | Handedness | Risk factors | Neuropsychological condition | Number of ASMs | Seizure frequency (/months) | Seizure type* | Seizure location* | Epilepsy type |
Preoperative imaging studies |
||
---|---|---|---|---|---|---|---|---|---|---|---|---|---|
Structural abnormality | Perfusion (ictal SPECT) | Metabolism (FDG-PET) | |||||||||||
1 | Female/38 | 9 (29) | Rt. | - | ID | 2 | 14 | FIAS | F3 (60), F4 (40) | FLE, Lt. | - | ↑Rt. F/T/P/BG | ↓Lt. F |
2 | Female/57 | 27 (30) | Rt. | - | - | 3 | 1.5 | FTBTCS | F8 (65), F7 (35) | TLE, Bilat. | Rt. HS | ↑Bilat. T | ↓Rt. T |
3 | Male/36 | 22 (14) | Rt. | Enceph. | - | 3 | 1.5 | FIAS | Sp1 (70), Sp2 (30) | TLE, Lt. | - | ↑Lt. T | ↓Lt. T |
4 | Female/30 | 27 (3) | Rt. | FC | - | 3 | 6.0 | FTBTCS | T7 (50), T8 (50) | TLE, Lt. | - | ↑Lt. T | ↓Rt. T |
5 | Male/26 | 14 (12) | Rt. | - | ID | 5 | 1.5 | FIAS | Sp2 (75), Sp1 (25) | TLE, Rt. | Rt. HS | - | ↓Rt. T |
6 | Male/15 | 8 (7) | Lt. | FC | ID | 4 | 30 | FTBTCS | C3 (50), C4 (50) | DEE | Lt. F EM | - | - |
7 | Female/25 | 20 (5) | Rt. | Enceph.; SE | - | 4 | 3.0 | FTBTCS | Sp2 (60), Sp1 (40) | TLE, Rt. | Rt. F DVA | ↑Rt. T/BG | ↓Rt. F/T/P |
8 | Male/35 | 21 (14) | Rt. | - | - | 3 | 1.0 | FIAS | Sp2 (100) | TLE, Rt. | Lt. HS | ↑Lt. F/T/P/BG | ↓Rt. F |
9 | Female/51 | 22 (29) | Rt. | - | - | 3 | 2.5 | FIAS | T8 (100) | TLE, Rt. | - | - | - |
10 | Male/46 | 36 (10) | Rt. | - | - | 4 | 1.5 | FIAS | T7/F7 (60), T8/F8 (40) | TLE, Bilat. | Bilat. O EM | ↑Lt. T | ↓Lt. T |
11 | Female/34 | 14 (20) | Rt. | FC | - | 3 | 4.0 | FIAS | T7/F3 (80), T8 (20) | TLE, Lt. | Lt. T EM, Lt. O CM | - | ↓Lt. T |
12 | Male/32 | 12 (20) | Rt. | Enceph. | - | 3 | 0.4 | FTBTCS | T7/F7 (67), T8/F8 (33) | FLE, Bilat. | - | ↑Rt. F/T | - |
13 | Male/15 | 3 (12) | Ambidex. | - | ID | 5 | 30 | FIAS | T7/F7 (67), T8/F8 (33) | DEE | Bilat. F EM | - | - |
14 | Female/32 | 15 (17) | Rt. | - | - | 3 | 1.5 | FIAS | Sp1 (60), Sp2 (40) | TLE, Bilat. | - | ↑Lt. T/BG | ↓Lt. T |
15 | Female/48 | 32 (16) | Rt. | - | Depression | 3 | 6.0 | FIAS | Sp2 (100) | TLE, Rt. | - | ↑Rt. T | ↓Rt. F/T/P |
16 | Female/49 | 39 (10) | Rt. | Enceph., SE | - | 5 | 1.5 | FIAS | Sp1 (60), Sp2 (40) | TLE, Bilat. | Bilat. HS | ↑Rt. T | ↓Bilat. T |
* Most common seizure characteristics recorded during scalp and sphenoidal video-electroencephalography monitoring.
ASM : antiseizure medication, SPECT : single-photon emission computerized tomography, FDG-PET : [18F]fluorodeoxyglucose positron emission tomography, Rt. : right, ID : intellectual disability, FIAS : focal impaired awareness seizure, FLE : frontal lobe epilepsy, Lt. : left, F : frontal, T : temporal, P : parietal, BG : basal ganglia, FBTCS : focal to bilateral tonic-clonic seizure, TLE : temporal lobe epilepsy, Bilat. : bilateral, HS : hippocampal sclerosis, Enceph. : encephalitis, FC : febrile convulsion, DEE : developmental and epileptic encephalopathy, EM : encephalomalacia, SE : status epilepticus, DVA : developmental venous anomaly, O : occipital, CM : cavernous malformation, Ambidex. : ambidextrous
Table 2.
Case | Number of electrodes, Rt./Lt. | Duration of monitoring (days) | Number of recorded seizures* | Ictal onset zone | Localization | Surgical intervention | Histopathology | Postop. follow-up (months) | Seizure outcome† | ASM adjustment |
---|---|---|---|---|---|---|---|---|---|---|
1 | 0/9 | 10 | 10 (0) | Lt. F (100) | Lt. F | Lt. F lobectomy | Non-specific | 16 | IV | No reduction |
2 | 4/4 | 8 | 6 (2) | Rt. MT (50), Lt. T (50) | Bilat. T | ANT-DBS | - | 16 | II | No reduction |
3 | 5/7 | 11 | 23 (0) | Lt. MT (96) Rt. MT (4) | Lt. T | Lt. ATL-AH | Non-specific | 13 | Ia | No reduction |
4 | 8/3; 8/5‡ | 9; 11‡ | 41 (32); 19 (8)‡ | Rt. MT (67), Lt. MT (33) | Bilat. T | - | - | - | - | - |
5 | 4/4 | 8 | >99 (8) | Rt. MT (100) | Rt. T | Rt. ATL-AH | HS, SBH | 17 | Ia | Reduction |
6 | 0/9 | 6 | 13 (0) | Lt. F (100) | Lt. F | Lt. F topectomy | FCD IIa | 12 | Ia | Reduction |
7 | 12/6 | 12 | 12 (4) | Lt. T/MT (58), Rt. T (42) | Bilat. T | - | - | - | - | - |
8 | 6/6 | 16 | 4 (0) | Rt. MT (100) | Rt. T | Rt. ATL | Non-specific | 10 | Ia | Reduction |
9 | 7/1 | 12 | 27 (1) | Rt. MT (100) | Rt. T | Rt. ATL-AH | FCD I | 4 | Ia | Reduction |
10 | 6/8 | 11 | 12 (0) | Lt. MT (58), Rt. MT (42) | Bilat. T | - | - | - | - | - |
11 | 3/7 | 7 | 13 (0) | Lt. T (85), Lt. MT (15) | Lt. T | Lt. ATL-AH | EM | 4 | Ia | No reduction |
12 | 11/0 | 13 | 3 (3) | Rt. F/OI (100) | Rt. F | Lt. F topectomy | FCD I | 5 | III | No reduction |
13 | 3/11 | 8 | >99 (100) | Lt. F/C (100) | Lt. F | Lt. F lobectomy | EM | 4 | Ia | Reduction |
14 | 7/9 | 12 | 9 (9) | Lt. MT (89), Rt. T (11) | Lt. T | - | - | - | - | - |
15 | 11/1 | 11 | 17 (0) | Rt. MT (76), Rt. T (24) | Rt. T | Rt. ATL-AH | HS, FCD IIIa | <3 | Ia | Reduction |
16 | 8/8 | 7 | 34 (0) | Lt. T/MT (56), Rt. T/MT (44) | Bilat. T | - | - | - | - | - |
SEEG : stereoelectroencephalography, Rt. : right, Lt. : left, Postop. : postoperative, ASM : antiseizure medication, F : frontal cortex, MT : mesial temporal region (amygdala and hippocampus), Bilat. : bilateral, T : temporal cortex, ANT-DBS : deep brain stimulation of the anterior nucleus of the thalamus, ATL-AH : anterior temporal lobectomy with amygdalohippocampectomy, HS : hippocampal sclerosis, SBH : subcortical band heterotopia (laminar heterotopia), FCD : focal cortical dysplasia, EM : encephalomalacia, OI : operculo-insular region, C : cingulate cortex
Table 3.
Study | Study population | Stereotactic instruments | Number of electrodes per case* | Morbidity† | Electrode misplacement |
Accuracy measures |
Time for electrode placement‡ (minutes) | |
---|---|---|---|---|---|---|---|---|
EPLE (mm) | TPLE (mm) | |||||||
Frame-based SEEG techniques | ||||||||
González-Martínez et al. [19] (2016) | Adult | Leksell | 13.3 (1367/103) | 0.2% (3.0%) | - | 1.1 | - | 26.5 (352) |
van der Loo et al. [43] (2017) | Adult | Leksell | 11.9 (902/76) | 0.2% (2.6%) | - | 1.5 | 2.9 | 11.4 (136) |
Dewan et al. [14] (2018) | Adult | Customized | 9.1 (137/15) | - | - | 1.4±1.0 | 3.4±2.7 | 24.0 (207) |
Yu et al. [45] (2018) | Adult | Customized | 6.5 (136/21) | - | - | 1.2 (0.8–1.8) | 4.5 (2.4–7.7) | - |
Bourdillon et al. [5] (2018) | Adult | Talairach | 12.6 (628/50) | 0.2% (5.0%) | 0.2% | - | 4.0±1.0 | 19.6 (246) |
D'Agostino et al. [12] (2020) | Adult | Customized | 14.0 (182/13) | 1.6% (23.1%) | - | 2.0 (1.2–2.9) | 5.0 (3.0–6.9) | 10.3 (144) |
Girgis et al. [17] (2020) | Adult | CRW | 3.6 (36/10) | - | - | - | 7.0 | - |
Zheng et al. [46] (2021) | All | Leksell | 6.4 (90/14) | 2.2% (14.3%) | - | 1.5±0.5 | 1.6±0.7 | 24.6 (153) |
Machetanz et al. [31] (2021) | All | BRW | 7.6 (91/12) | - | - | 1.5±0.6 | 1.5±0.8 | 15.1 |
Kogias et al. [28] (2022) | All | Leksell | 9.1 (136/15) | 0.7% (6.7%) | - | 0.6 | 1.5 | - |
This study | All | Leksell | 11.8 (201/17) | 0.5% (5.9%) | 1.0% | - | - | 13.0 (162) |
Image-guided SEEG techniques | ||||||||
Nowell et al. [34] (2014) | Adult | Medtronic | 8.5 (187/22) | 0.5% (4.5%) | 6.4% | - | 3.7±2.2 | 16.1 (137) |
Hou et al. [21] (2016) | Adult | Medtronic | 4.8 (173/36) | 1.2% (5.6%) | - | - | 2.0±1.0 | 19.4 |
Verburg et al. [44] (2016) | Adult | Brainlab | 12.7 (89/7) | - | - | - | 3.5 | - |
Roessler et al. [36] (2016) | Adult | Brainlab | 9.7 (58/6) | - | - | 1.4±1.2 | 3.2±2.2 | 11.8 (115) |
Budke et al. [6] (2018) | Pediatric | Brainlab | 7.4 (111/15) | 0.9% (6.7%) | - | 3.6±1.8 | 2.9±1.5 | 15.7 (90) |
Sharma et al. [37] (2019) | Pediatric | Medtronic | 10.9 (218/20) (n=6) | - | - | 4.5 (2.8–6.1) | 5.5 (4.0–6.4) | - |
Kim et al. [26] (2020) | All | Medtronic | 7.1 (177/25) | - | - | - | - | 24.0 (173) |
Chen et al. [11] (2020) | Pediatric | Medtronic | 4.5 (81/18) | - | - | 2.0±0.6 | 2.6±0.6 | - |
Girgis et al. [17] (2020) | Adult | Medtronic | 3.5 (45/13) | - | 17.8% | - | 11.0 | - |
Ladisich et al. [29] (2021) | Adult | Brainlab | 10.5 (220/21) | 0.5% (4.8%) | 0.5% | 3.2±2.4 | 2.7±2.0 | - |
Vakharia et al. [41] (2021) | Adult | Medtronic | 10.5 (168/16) | - | - | 1.2±0.1 | 1.2±0.2 | 9.1 (202) |
Song et al. [38] (2021) | Adult | Brainlab | 7.4 (141/19) | - | - | 2.0±0.5 | 2.5±0.8 | 14.2 |
Joris et al. [23] (2023) | All | Brainlab | 8.5 (94/11) | 1.1% (9.1%) | 1.1% | 4.2±2.5 | 4.1±2.5 | 18.6 (158) |
Robot-assisted SEEG techniques | ||||||||
Cardinale et al. [8] (2013) | All | Neuromate | 13.3 (1567/118) | 0.2% (3.2%) | - | 0.9 (0.6–1.4) | 2.0 (1.4–3.0) | 24.1 (320) |
González-Martínez et al. [19] (2016) | Adult | ROSA | 12.3 (1245/101) | 0.3% (4.0%) | - | 1.2 (0.8–1.8) | 1.7 (1.2–2.3) | 10.5 (130) |
Dorfer et al. [15] (2017) | Adult | iSYS1 | 5.8 (93/16) | 1.1% (6.3%) | - | 1.3 | 1.5 | 15.7 |
Cardinale et al. [9] (2017) | Adult | Neuromate | 15.9 (127/8) | - | - | 0.6 (0.3–0.9) | 1.5 (1.1–2.4) | - |
Candela-Cantó et al. [7] (2018) | Pediatric | Neuromate | 11.7 (164/14) | 1.2% (14.3%) | - | 1.6 (1.0–2.3) | 1.8 (1.2–2.6) | 35.4 (414) |
Bourdillon et al. [5] (2018) | Adult | Neuromate | 11.3 (565/50) | - | 0.2% | - | 1.2±0.4 | 22.1 (250) |
McGovern et al. [32] (2019) | Pediatric | ROSA | 12.4 (794/64) | 0.1% (1.5%) | - | - | - | 9.6 |
Sharma et al. [37] (2019) | Pediatric | ROSA | 10.9 (218/20) (n=14) | - | - | 1.1 (0.7–1.6) | 0.7 (0.5–1.0) | - |
Spyrantis et al. [39] (2019) | All | ROSA | 9.0 (171/19) | - | - | 1.9±1.1 | 2.6±1.7 | - |
Kim et al. [26] (2020) | All | ROSA | 10.2 (255/25) | - | - | 1.4±0.8 | 3.1±2.0 | 12.4 (126) |
Bottan et al. [4] (2020) | Adult | Neuromate | 2.4 (98/41) | 1.5±1.2 | 2.3±1.5 | |||
Zheng et al. [46] (2021) | All | SINO | 10.7 (203/19) | 2.0% (21.1%) | - | 1.4±0.5 | 1.5±0.4 | 13.7 (127) |
Machetanz et al. [31] (2021) | All | ROSA | 8.6 (129/15) | 0.8% (6.7%) | - | 0.7±0.5 | 1.6±0.8 | 9.1 |
Bonda et al. [3] (2021) | Pediatric | ROSA | 11.3 (261/23) | - | - | - | 4.5±3.5 | 6.6 |
Vakharia et al. [41] (2021) | Adult | iSYS1 | 10.0 (160/16) | - | - | 1.1±0.1 | 1.6±0.2 | 6.4 (176) |
Kandregula et al. [24] (2021) | Adult | Neuromate | 12.8 (294/23) | 0.7% (8.7%) | - | 1.5 (1.1–2.3) | 2.3 (1.5–3.5) | - |
Hines et al. [20] (2023) | Adult | Neuromate | 13.8 (525/38) | - | - | 1.2 (0.8–1.8) | 2.4 (1.6–3.5) | - |
Dedrickson et al. [13] (2023) | Adult | ExcelsiusGPS | 11.0 (55/5) | - | - | 1.6±1.2 | - | 14.2 (156) |
A literature search on PubMed covered publications from 2013 to 2023. Selected studies that specifically reported clinical data on the safety, accuracy, and/or time efficiency of various stereotactic techniques were selectively reviewed and summarized, with duplicated data removed. Data are presented as either counts, percentages per electrode (per case), medians (interquartile range), or mean±standard deviation, depending on availability, unless noted otherwise. Some estimates are derived from calculations based on reported values.