Abstract
Background
Methods
Results
Notes
Funding
This research was supported by the Basic Science Research Program through the National Research Foundation of Korea (NRF), funded by the Ministry of Education (NRF-2021R1I1A3040332). This research was supported by the Basic Science Research Program through the NRF, funded by the Ministry of Education (IRIS RS-2023-00238572). This study was supported by a NRF grant funded by the Korean government (MSIT) (NRF-2021R1F1A1062363). This research was supported by funding from the Waldemar von Frenckell Foundation (grant no 4706562) and the Finnish Society of Sciences and Letters (grant no 4709946).
Data Availability
The data presented in this study are available on reasonable request from the corresponding author.
Author Contributions
Soo Hee Lee (Data curation; Funding acquisition; Investigation; Methodology; Software; Validation; Visualization; Writing – review & editing)
Kyeong-Eon Park (Data curation; Investigation; Methodology; Software; Validation; Visualization; Writing – review & editing)
Kibaek Eum (Data curation; Investigation; Methodology; Writing – review & editing)
Yeran Hwang (Data curation; Investigation; Methodology; Validation; Writing – review & editing)
Seong-Ho Ok (Conceptualization; Funding acquisition; Resources)
Gyujin Sim (Data curation; Formal analysis; Investigation; Methodology; Software; Validation; Visualization; Writing – review & editing)
Dumidu Perera (Data curation; Investigation; Methodology; Writing – original draft)
Henri K.M. Ravald (Data curation; Investigation; Methodology; Writing – original draft)
Youngho Park (Resources; Writing – review & editing)
Susanne K. Wiedmer (Conceptualization; Formal analysis; Funding acquisition; Project administration; Supervision; Writing – original draft; Writing – review & editing)
Ju-Tae Sohn (Conceptualization; Formal analysis; Funding acquisition; Methodology; Project administration; Supervision; Validation; Writing – original draft; Writing – review & editing)
References
Fig. 1.
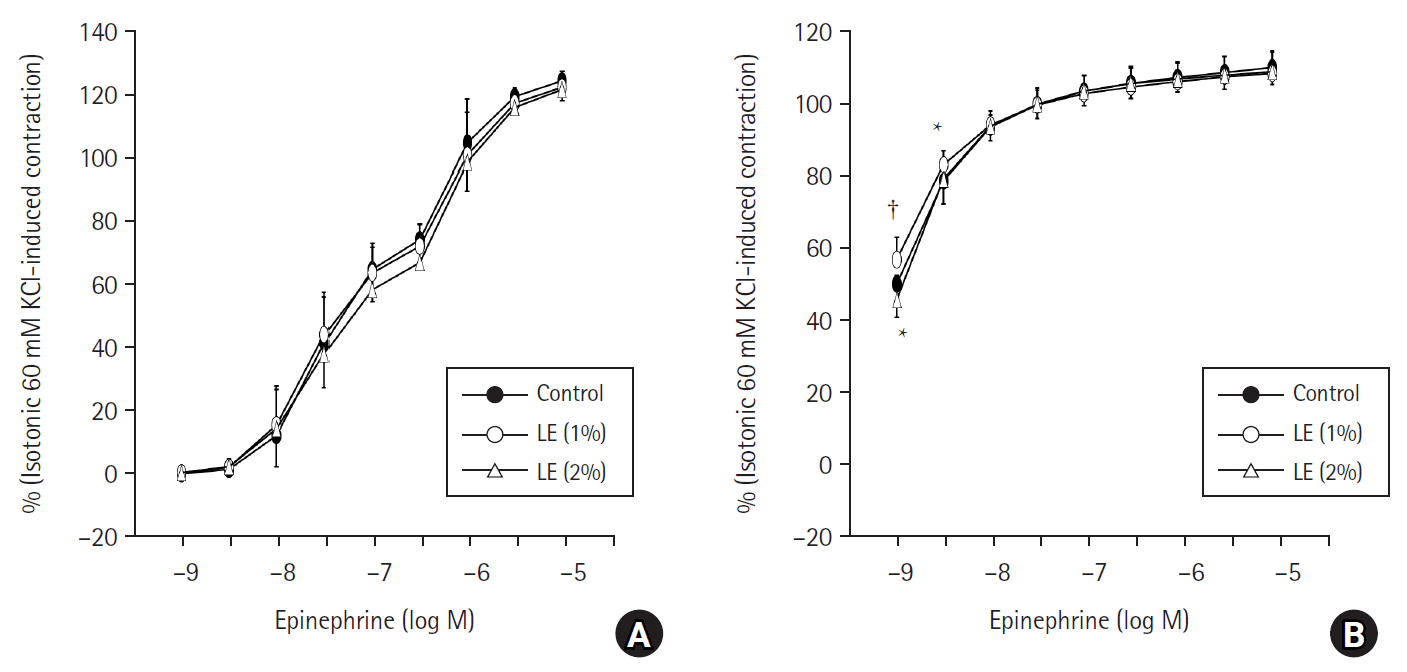
Fig. 2.
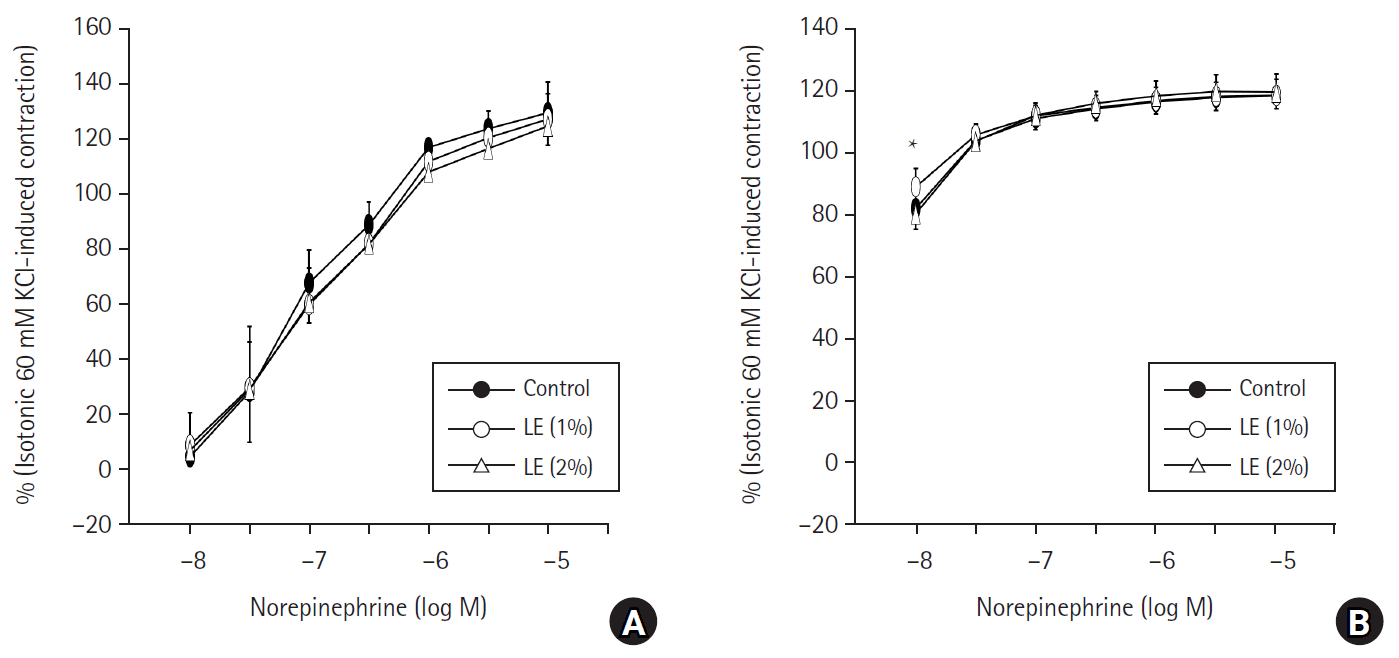
Fig. 3.
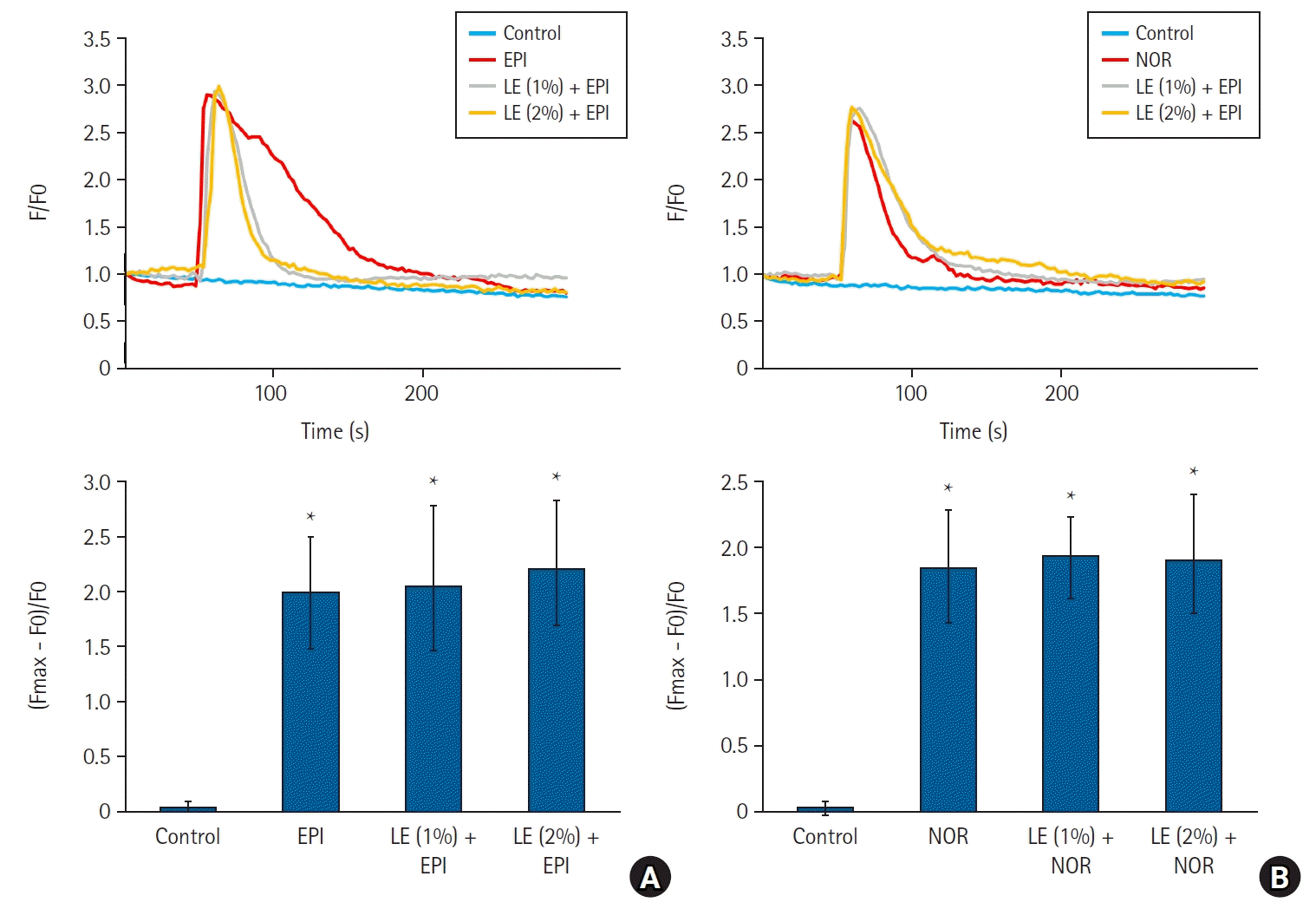
Fig. 4.
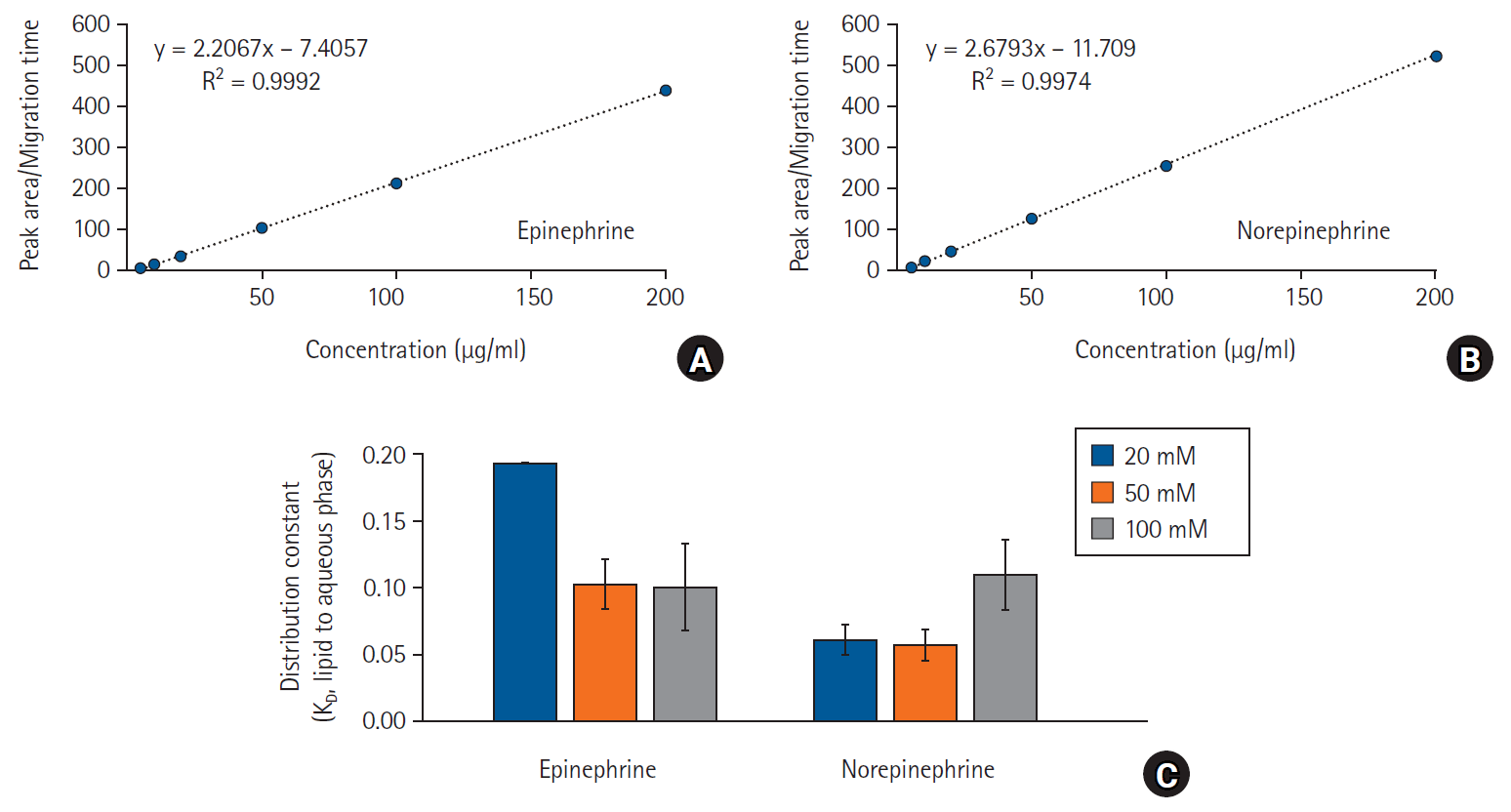
Table 1.
Drug | log P* | pKa† | NIF (%)‡ at pH 7.4 | NIF (%)‡ at pH 7.3 | NIF (%)‡ at pH 7.2 | NIF (%)‡ at pH 7.1 |
---|---|---|---|---|---|---|
EPI | −1.37 | 8.59 | 6.06 | 4.88 | 3.91 | 3.13 |
NOR | −1.24 | 8.58 | 6.20 | 4.99 | 4.00 | 3.21 |
*log P: log octanol/water partition coefficient from PubChem (https://pubchem.ncbi.nlm.nih.gov/). †pKa: negative logarithm of the acid dissociation constant (Ka) from PubChem (https://pubchem.ncbi.nlm.nih.gov/). ‡NIF (%): non-ionized fraction of total drug (EPI and NOR) is calculated using Henderson-Hasselbalch equation. EPI: epinephrine; NOR: norepinephrine.