Abstract
The recurrent laryngeal nerve is a bilateral branch of the vagus nerve that is mainly associated with the motor innervation of the intrinsic muscles of the larynx. Despite its bilateral distribution, the right and left recurrent laryngeal nerves display unequal length due to embryological processes related to the development of the aortic arches. This length asymmetry leads to theories about morphological compensations to provide symmetrical functions to the intrinsic muscles of the larynx. In this study we investigated the developmental and cross-sectional morphometrics of the recurrent laryngeal nerves in human fetuses. Fifteen stillbirth fetuses donated to anatomical and medical research were used for investigation. Fetuses had intrauterine age ranging from 30 to 40 weeks estimated by biometry methods. Specialized anatomical dissection of the visceral block of the neck was performed to prepare histological samples of the recurrent laryngeal nerves in its point of contact with the larynx, and morpho-quantitative techniques were applied to evaluate the epineurium and perineural space of the recurrent laryngeal nerves. No statistical difference in the cross-sectional morphology of the epineurium and perineural space between right and left recurrent laryngeal nerves intra-individually was confirmed, however, we found evidence that these structures are under greater development in the left recurrent laryngeal nerve during 30 to 40 weeks of intrauterine life. Our data suggest that the nerves are under morphological development that possibly set the stage for accommodation of larger diameter and myelinization of the left recurrent laryngeal nerve during post-natal life.
The human larynx was once compared to a wind instrument [1]. Vocal sounds were thought to be produced by a current of air passing the resistance of the vocal cords and causing them to vibrate in mechanical fashion. According to this theory, the nervous system would function only to maintain the vocal cord tension required to generate a particular sound pitch. Nonetheless, stroboscopic observations [2] have long supported the concept that the vocal cords actively vibrate during the phonation process instead of assuming a passive vibratory behaviour dependent of infraglotic pressure.
Most intrinsic muscles of the human larynx, except cricothyroid muscles, are innervated by the recurrent laryngeal nerves (RLNs). The RLN is described as a branch of the vagus nerve, the cranial nerve X that carries motor, sensory and parasympathetic fibers to the larynx. Despite having the same distribution on both sides of the body, each RLN loops around two different structures at two different body levels, an arrangement that is correlated with the development of the aortic arches in the embryo [3]. The right recurrent laryngeal nerve (R-RLN) loops inferior to the right subclavian artery at the T1–2 vertebral level, while the left recurrent laryngeal nerve (L-RLN) loops inferior to the aortic arch at the T4–5 vertebral level. After looping, the RLNs ascend on the tracheoesophageal groove, in contact with posteromedial aspect of the thyroid gland, supplying the trachea, esophagus, and the majority of the intrinsic laryngeal muscles [4].
The lengths of the R- and L-RLN differ since they wind around two distinct structures of the body. Adult individuals have the R-RLN reported on an average of 4 to 9 cm, while the L-RLN have been described with lengths of about 6 to 13 cm [5-7]. Considering that intrinsic muscles of the larynx are responsible for changing the length, tension, and vibration of the vocal folds in a symmetrical way; would the differences in the length of the R- and L-RLNs be prohibitive for the simultaneous arrival of impulses to the vocal cords? or would the nervous system be equipped with morphological and/or physiological mechanisms that allow for synchronized muscle activation?
Although studies have considered slight delays in the activation of the intrinsic muscles of the larynx due to morphological disparities in the RLNs [6, 8, 9], there is also evidence that nerve components in the R- and L-RLN differ allowing simultaneous activation of the intrinsic muscles of the larynx [10]. Specifically, the myelin sheath and transverse sectional area of the L-RLN are reported to be larger to compensate the length difference between both nerves [11, 12].
In this study, we have investigated the morphological features of the RLNs during 30 to 40 weeks of intrauterine development. We found evidence that the epineurium and the perineural space of the L-RLN are under temporal development that may set the stage for accommodation of larger diameter of the L-RLN during post-natal life.
Experiments were conducted on 15 human fetuses from the anatomical collection of the Department of Morphology at the Federal University of Espirito Santo (UFES). The stillbirth fetuses were referred to the Department of Morphology-UFES from maternity hospitals located in Vitoria municipality according to institutional operational procedures for family donation and Brazilian Federal Law 8.501/92. Specimens had no signs of fetal anomaly and were fixed and maintained in 4% formalin solution. The age of the specimens ranged from 30–40 weeks (Table 1) estimated by fetal biometry methods [13-15].
This research was conducted in accordance with the principles embodied in the Declaration of Helsink and local statutory requirements. All experiments were approved by the Institutional Research Ethics Board involving human subjects and registered in the National Research Ethics Commission - CEP/CONEP system (Plataforma Brasil, protocol number 3.565.100).
The presence of the RLNs was verified by specialized dissection of the root of the neck on the right side and the superior mediastinum on the left side. After evidencing the origin of the nerves, the visceral layer of the neck was carefully isolated anteriorly to the retropharyngeal space and prevertebral fascia by blunt dissection. Two transverse incisions were performed to collect the visceral block of the neck. The first incision was made at the level of the thyrohyoid membrane and the second at the level of the 3rd tracheal ring, the resulting tissue block contained the pharynx, larynx, trachea, and esophagus. Once the tissue block was removed, a median longitudinal incision was performed to separate the right and left halves of the specimens with respective RLNs in situ.
Left and right samples extracted from the tissue block were then dehydrated in a progressive series of alcohols 70%–100% and 3x xylene baths prior to incubation in paraffin. The specimens were systematically and consistently supported during the incubation process to guarantee longitudinal orientation through the paraffin blocks. After, microtome sections of 6 µm were performed in axial orientation starting from the inferior surface of the specimen. Sections were collected in a semi-serial manner of 1 each 10 slices, placed in histological slides and deparaffinized in a laboratory drying oven at 60°C before Mallory’s [16] trichrome staining. Finally, slides were coverslip over mounting medium before microscopic analysis.
Image acquisition and analysis were performed with a Leica DM500 microscope equipped with a camera and LAS EZ 3.4.0 computer program. Slides showing the inferior border of the cricoid cartilage where the RLN was topographically external to the cricoid cartilage and internal to the cricopharyngeus muscle were selected to perform the morphometry of the R- and L-RLNs (Fig. 1). Three to five sections from each side of the specimen at the aforementioned level were randomly selected to measure the cross-sectional area of the epineurium and the perineural space. Morphometric quantification of the cross-sectional area was obtained by digitally outlining the structures in calibrated photomicrographs using ImageJ (National Institutes of Health) and charting the results in Excel for Microsoft 365®. Specimens presenting discontinuity of the perineural epithelium or distorted perineural space were excluded from the data analysis. The linear eccentricity of the perineural space was calculated by e=c/a, and excluded from analysis if e>0.7.
Mean morphometric parameters of each specimen were calculated and statistical analysis was performed with the data analysis package for Excel (statistiXL, version 1.8). F-test was used to verify normal distribution of morphometrics and paired t-test to compare intra-individual differences between R- and L-RLNs morphometry. Linear regression analysis and Pearson’s correlation were generated to determine the relationship between age and morphometric parameters. The strength of relationship was determined none if r<0.3, weak if 0.3<r<0.49, moderate if 0.5<r<0.69 and strong if r>0.7. Changes were considered statistically significant if P<0.05.
The RLN was found in all 15 fetuses in the tracheoesophageal groove with its typical trajectory pattern. The R-RLN arose from the right vagus nerve in the root of the neck, approximately at the T1–2 vertebral level, and looped around the right subclavian artery before ascending into the tracheoesophageal groove (Fig. 2A). The L-RLN originated in the superior mediastinum as a detachment of the left vagus nerve, approximately at the T4–5 vertebral level, and curved inferiorly to the aortic arch immediately lateral to the ductus arteriosus (Fig. 2B).
The RLN gained laryngeal territory after crossing the inferior border of the cricoid cartilage (Fig. 3A). The epineurium of our RLN samples was easily distinguished from the rest of connective tissue at the examined level. We observed it as a dense reticular connective tissue surrounding the nerve fascicles provided of 2 to 4 vasa nervorum in its morphology (Fig. 3B). The minimal cross-section area of the epineurium was found in the R-RLN of a 33–34-week fetus (13,860.98±1,910.19 µm2), whereas the largest cross-section area recorded from a L-RLN given by a 38–39-week fetus (96,852.97±9,729.94 µm2). Eight out of fifteen fetuses had the epineurium cross-sectional area larger on its left side (Fig. 3C, I), however these differences were not statistically significant intra-individuals (P=0.74). Table 1 displays fetal epineurium morphometric parameters.
The neural compartment located among axons, supporting cells, and circumscribed by the deeper portion of the epineurium, was defined as the perineural space (Fig. 3B). Minimum values for the perineural space were found in a R-RLN of a 33–34-week fetus (10,421.85±1,508.21 µm2), in addition to maximum values recorded to R-RLN of a 38–39-weeks fetus (71,187.86±3,867.52 µm2). The full sample comparison showed that the perineural space of ten out of fifteen fetuses was larger on its right side (Fig. 3C, II), however, the differences were not significant intra-individuals (P=0.54). Table 2 shows the morphometric parameters of perineural spaces.
The temporal function of our morphometric analysis revealed that the L-RLN is experiencing development beyond the R-RLN. Our results indicated a linear relationship between age and morphometric parameters of R- and L-RLN. All variables showed a positive values association with r values ranging from 0.31 to 0.74 (Fig. 4). The R-RLN displayed a weak strength of relationship (r=0.31) for the development of the epineurium over the time, and that correlation was not statistically significant (P=0.26) (Fig. 4A, I). However, the development of the epineurium of the L-RLN exhibited a strong strength of relationship (r=0.74) with age and significant correlation (P<0.01) (Fig. 4A, II). The development of the perineural space presented a moderate strength of relationship (r=0.50) with a tendency to significant correlation (P=0.06) by age in the R-RLN (Fig. 4B, I), and the data from the L-RLN indicated a moderate strength of relationship (r=0.67) with significant correlation (P<0.01) of the perineural space during development (Fig. 4B, II).
Over the past century, studies about larynx innervation and function have sparked curiosity and controversy. Undoubtedly, the length asymmetry between the R- and L-RLNs added to the symmetric muscle function seems to be a driving force for different hypotheses and investigations. Our study has examined the RLN of human fetuses during an important developmental period. The fetal age from 30 to 40 weeks falls into the third gestational trimester, a crucial time for maturation and growth of all systems [17]. According to the World Health Organization, a stillbirth happens when fetal loss occurs anytime after 28 weeks towards the end of pregnancy [18]. There are risk factors to occurrence of a stillbirth, however, a large percentage of cases remains unknown even after autopsy examination [19]. We did not find signs of fetal anomaly or malformation in our post-mortem analysis.
An interesting finding in our study was that although the cross-sectional morphometric parameters of the RLN do not show intra-individual changes, our data indicates that the L-RLN is experiencing accentuated developmental changes compared with the R-RLN during the 30 to 40 weeks of intrauterine life. Whether this observed development has been carried out earlier than 30 weeks or continues after 40 weeks is still an open question for future investigations.
Nonetheless, a question that may arise from our dataset is: if there were no variations between each specimen, how come the L-RLN is experiencing greater development? The main explanation for this finding is that intra-individual analysis was less sensitive to detecting differences because it focused on changes within the same individual, which was relatively small compared to differences between individuals. Contrarily, in the inter-individual analysis to verify the development of the RLNs over time, we compared different individuals, where variations among them were larger and perhaps more sensitive in the statistical method. The nature of our data required methodology to approach variability within and between individuals, consequently, the intra-individual approach yields no significant results, while the inter-individual shows statistically significant results. We trust this novel morphological dataset adds value to the literature and may collaborate to fully understand the complex postnatal symmetrical functions of the larynx and RLNs. Future studies with increased female samples may be beneficial to investigate morphometric and developmental differences between sexes, the number of female fetuses in our study was low for compelling comparative statistical analyses.
During the study design, a challenging point was to define the location for sample analysis. In its ascending journey, deeper to the cricopharyngeus muscle while crossing the inferior border of the cricoid cartilage, the RLN is perceptible as a single nerve trunk for a few millimeters regardless of reported anatomical variations such as Galen’s anastomosis [20], the arytenoid plexus or cricoid communications [21]. Therefore, the RLN fibers destined for other neck structures were not present at the examined level and our cross-sectional morphometry represents the stem of the RLN solely intended for the vocal folds and the intrinsic muscles of the larynx. A similar approach was found in two studies investigating the morphometry of the RLN in human specimens [22, 23].
Throughout the embryo development, the heart descends while the neck elongates. Since the L-RLN winds around the ductus arteriosus, the nerve is drawn caudally to the superior mediastinum [17]. On the other hand, no vascular structures drag the R-RLN into the thoracic cavity, as in the right side of the embryo the distal part of the fifth and sixth aortic arches involute, leaving the nerve free to migrate into the neck, hooking around the right subclavian artery, a structure derived from the fourth aortic arch [5, 17]. In addition, the structure and function of the larynx changes drastically during development. After birth, neonates are able to guard lower airways, regulate respiration airflow, and vocalize by laryngeal mechanisms that require proper innervation [24]. Morphometric studies in adult humans have demonstrated that the RLN have a significantly greater number of myelinated fibres than unmyelinated [25]. The initial myelination process in the vagus nerves start early at the 14 weeks of development [26], however, visualization of the myelin sheath on light microscopy may require numerous myelin lamellae [27]. In our study was not possible to differentiate myelinated from unmyelinated fibers of the RLN due to method limitations or relatively few myelin lamellae during 30–40 weeks of intrauterine development. We suggest future studies with appropriate specific stains to detect myelinated fibers, or more advanced techniques such as transmission electron microscopy, or immunohistochemistry to identify myelin basic protein at the sectioned level to unveil early myelin lamella and myelinization processes of the RLNs during this period of development.
The epineurium is defined as the dense irregular connective tissue enveloping peripheral nerves. It usually encircles the surface of a nerve and give passage to blood vessels responsible for nerve supply know as vasa nervorum. Smaller branches of these vessels ultimately dive into the perineurium to communicate with a network of fine vessels within the endoneurium, forming an intrinsic system of vascular plexuses [28]. Not only blood vessels navigate thought the epineurium but also lymphocytes and fibroblasts are key elements found in the epineural structure. Given the importance of those elements for development, maintenance, and tissue repair; one could expect that the L-RLN of the fetuses would show a thicker epineurium than the R-RLN. However, our results indicate that the epineurium area of R- and L-RLN is equally thick among single individuals. One explanation for these findings is that at this stage of development, the functionality of the larynx is modest. The RLNs only connect the central nervous system to the larynx without the need for sophisticated functions, therefore it is not astonishing that similar cross-sectional morphology was found in both RLN intra-individuals. However, plotting the morphological results in a time dependent function among different individuals, we observed that the epineurium of the L-RLN is undergoing major development compared to the R-RLN, and these results endorse the different morphological traits of the RLNs found intra-individuals postnatally [5, 6, 23].
Nerve axons are supported by Schwann cells, fibroblasts, capillaries, and scattered mast cells [29]. The cell matrix associated with the axons is referred to as the endoneurium, and the connective tissue surrounding the endoneurium in circular fashion is described as perineurium [30]. The internal surface of the perineurium is covered by squamous-like epithelial cells. This continuous layer of cell membrane covers the entire peripheral nervous system and outlines the perineural space. The functional significance of this membrane and space is that it serves as a diffusion barrier with protection mechanisms for the peripheral nerves, in addition to offer room and milieu for myelinization in developing axons [26, 31, 32]. Our results have shown that the perineural space of the R- and L-RLN are similar intra-individually in fetuses, however, we found tendency for age correlation in the development of the perineural space of the R-RLN, and evidence of significant development of the perineural space of the L-RLNs over the time. These findings are in line with what is expected of postnatal morpho-functional differences [8, 11, 25] and explained by the same rationale discussed anteriorly.
In conclusion, our results suggest that the L-RLN is experiencing morphological changes beyond the R-RLN during the period of 30 to 40 weeks of intrauterine life. This cross-sectional development may represent the structural changes necessary to accommodate the variation of length between R- and L-RLN. Possibly, the temporal development of the epineurium and the perineural space of the L-RLN as reported here endorses morphological basis allowing for larger cross-section of the L-RLN found in post-natal life.
Acknowledgements
We thank and appreciate the research support from the histology and anatomy technicians in the Department of Morphology at the Federal University of the Espirito Santo. We acknowledge and sincerely thank the families of the donors for their altruistic act in favor of medical science and anatomical research. These donors and their families deserve our highest gratitude.
Notes
References
1. Damste PH. 1965; The larynx as a wind-instrument. Pract Otorhinolaryngol (Basel). 27:94–5. DOI: 10.1159/000274637. PMID: 14302934.
2. Saito S, Fukuda H, Kitahara S, Kokawa N. 1978; Stroboscopic observation of vocal fold vibration with fiberoptics. Folia Phoniatr (Basel). 30:241–4. DOI: 10.1159/000264132. PMID: 730090.
3. Gardner ED, O'Rahilly R, Müller F. Gardner-Gray-O'Rahilly anatomy: a regional study of human structure. 5th ed. Saunders;1986.
4. Dalley AF, Agur AMR. Moore's clinically oriented anatomy. 9th ed. Wolters Kluwer Health;2021.
5. Maranillo E, de Blas CS, Górriz MC, Quinones S, Verdú E, Quer M, León X, Vázquez T, Sañudo JR, Konschake M. 2021; Comparative study of the length of human laryngeal nerves and their variations: functional and clinical considerations. Eur J Anat. 25:653–63.
6. Prades JM, Dubois MD, Dumollard JM, Tordella L, Rigail J, Timoshenko AP, Peoc'h M. 2012; Morphological and functional asymmetry of the human recurrent laryngeal nerve. Surg Radiol Anat. 34:903–8. DOI: 10.1007/s00276-012-0999-7. PMID: 23150169.
7. Valenzuela-Fuenzalida JJ, Baeza-Garrido V, Navia-Ramírez MF, Cariseo-Ávila C, Bruna-Mejías A, Becerra-Farfan Á, Lopez E, Orellana Donoso M, Loyola-Sepulveda W. 2023; Systematic review and meta-analysis: recurrent laryngeal nerve variants and their implication in surgery and neck pathologies, using the anatomical quality assurance (AQUA) checklist. Life (Basel). 13:1077. DOI: 10.3390/life13051077. PMID: 37240722. PMCID: PMC10221453.
8. Atkins JP Jr. 1973; An electromyographic study of recurrent laryngeal nerve conduction and its clinical applications. Laryngoscope. 83:796–807. DOI: 10.1288/00005537-197305000-00015. PMID: 4702478.
9. Pascual-Font A, Merchán A, Maranillo E, Brillas A, Sañudo JR, Valderrama-Canales FJ. 2006; Morphometry of the recurrent laryngeal nerves of the rat. Acta Otorrinolaringol Esp. 57:435–40. Spanish. DOI: 10.1016/S0001-6519(06)78744-1. PMID: 17228641.
10. Patel RR, Awan SN, Barkmeier-Kraemer J, Courey M, Deliyski D, Eadie T, Paul D, Švec JG, Hillman R. 2018; Recommended protocols for instrumental assessment of voice: American Speech-Language-Hearing Association expert panel to develop a protocol for instrumental assessment of vocal function. Am J Speech Lang Pathol. 27:887–905. DOI: 10.1044/2018_AJSLP-17-0009. PMID: 29955816.
11. Harrison DF. 1981; Fibre size frequency in the recurrent laryngeal nerves of man and giraffe. Acta Otolaryngol. 91:383–9. DOI: 10.3109/00016488109138519. PMID: 7023176.
12. Shin T, Rabuzzi DD. 1971; Conduction studies of the canine recurrent laryngeal nerve. Laryngoscope. 81:586–96. DOI: 10.1288/00005537-197104000-00010. PMID: 5553125.
13. March MI, Warsof SL, Chauhan SP. 2012; Fetal biometry: relevance in obstetrical practice. Clin Obstet Gynecol. 55:281–7. DOI: 10.1097/GRF.0b013e3182446e9b. PMID: 22343244.
14. Streeter GL. 1920; Weight, sitting height, head size, foot length, and menstrual age of the human embryo. Carnegie Inst Wash. 11:143–79.
15. Kinoshita H, Umezawa T, Omine Y, Kasahara M, Rodríguez-Vázquez JF, Murakami G, Abe S. 2013; Distribution of elastic fibers in the head and neck: a histological study using late-stage human fetuses. Anat Cell Biol. 46:39–48. DOI: 10.5115/acb.2013.46.1.39. PMID: 23560235. PMCID: PMC3615611.
16. Mallory FB. 1900; A contribution to staining methods: I. A differential stain for connective-tissue fibrillae and reticulum. II. Chloride of iron haematoxylin for nuclei and fibrin. III. Phosphotungstic acid haematoxylin for neuroglia fibres. J Exp Med. 5:15–20. DOI: 10.1084/jem.5.1.15. PMID: 19866932. PMCID: PMC2117995.
17. Sadler TW. Langman's medical embryology. 15th ed. Wolters Kluwer;2024.
18. World Health Organization. c2024. Stillbirth [Internet]. World Health Organization;Available from: https://www.who.int/health-topics/stillbirth. cited 2024 Apr 4.
19. Aminu M, Bar-Zeev S, van den Broek N. 2017; Cause of and factors associated with stillbirth: a systematic review of classification systems. Acta Obstet Gynecol Scand. 96:519–28. DOI: 10.1111/aogs.13126. PMID: 28295150. PMCID: PMC5413831.
20. Naidu L, Lazarus L, Partab P, Satyapal KS. 2014; Laryngeal nerve "anastomoses". Folia Morphol (Warsz). 73:30–6. DOI: 10.5603/FM.2014.0005. PMID: 24590520.
21. Henry BM, Pękala PA, Sanna B, Vikse J, Sanna S, Saganiak K, Tomaszewska IM, Tubbs RS, Tomaszewski KA. 2017; The anastomoses of the recurrent laryngeal nerve in the larynx: a meta-analysis and systematic review. J Voice. 31:495–503. DOI: 10.1016/j.jvoice.2016.11.004. PMID: 27939121.
22. Ellwanger JH, da Costa Rosa JP, dos Santos IP, da Rosa HT, Jotz GP, Xavier LL, de Campos D. 2013; Morphologic evaluation of the fetal recurrent laryngeal nerve and motor units in the thyroarytenoid muscle. J Voice. 27:668–73. DOI: 10.1016/j.jvoice.2013.07.004. PMID: 24128892.
23. Jotz GP, de Campos D, Rodrigues MF, Xavier LL. 2011; Histological asymmetry of the human recurrent laryngeal nerve. J Voice. 25:8–14. DOI: 10.1016/j.jvoice.2009.06.007. PMID: 20083375.
24. Harding R. 1984; Function of the larynx in the fetus and newborn. Annu Rev Physiol. 46:645–59. DOI: 10.1146/annurev.ph.46.030184.003241. PMID: 6370121.
25. Fleming JC, Gibbins N, Ingram PJ, Harries M. 2011; An anatomical study of the myelination of human laryngeal nerves. J Laryngol Otol. 125:1263–7. DOI: 10.1017/S0022215111001939. PMID: 21854693.
26. Woźniak W, O'Rahilly R. 1981; Fine structure and myelination of the developing human vagus nerve. Acta Anat (Basel). 109:218–30. DOI: 10.1159/000145387. PMID: 7257721.
27. Tanaka S, Mito T, Takashima S. 1995; Progress of myelination in the human fetal spinal nerve roots, spinal cord and brainstem with myelin basic protein immunohistochemistry. Early Hum Dev. 41:49–59. DOI: 10.1016/0378-3782(94)01608-R. PMID: 7540130.
28. Standring S, Gray H. Gray's anatomy: the anatomical basis of clinical practice. 42nd ed. Elsevier;2021. p. 1588.
29. Lanigan LG, Russell DS, Woolard KD, Pardo ID, Godfrey V, Jortner BS, Butt MT, Bolon B. 2021; Comparative pathology of the peripheral nervous system. Vet Pathol. 58:10–33. DOI: 10.1177/0300985820959231. PMID: 33016246.
30. Brown IS. 2016; Pathology of perineural spread. J Neurol Surg B Skull Base. 77:124–30. DOI: 10.1055/s-0036-1571837. PMID: 27123388. PMCID: PMC4846404.
31. Crowe TP, Greenlee MHW, Kanthasamy AG, Hsu WH. 2018; Mechanism of intranasal drug delivery directly to the brain. Life Sci. 195:44–52. DOI: 10.1016/j.lfs.2017.12.025. PMID: 29277310.
32. Shanthaveerappa TR, Bourne GH. 1966; Perineural epithelium: a new concept of its role in the integrity of the peripheral nervous system. Science. 154:1464–7. DOI: 10.1126/science.154.3755.1464. PMID: 5921613.
Fig. 1
Diagram of the external anatomy of the larynx, trachea, pharyngeal muscles, and esophagus in lateral view. The plane of the study for histological preparations is indicated in pink. Note that the cricopharyngeus muscle covers the recurrent laryngeal nerve in its final trajectory within the tracheoesophageal groove.
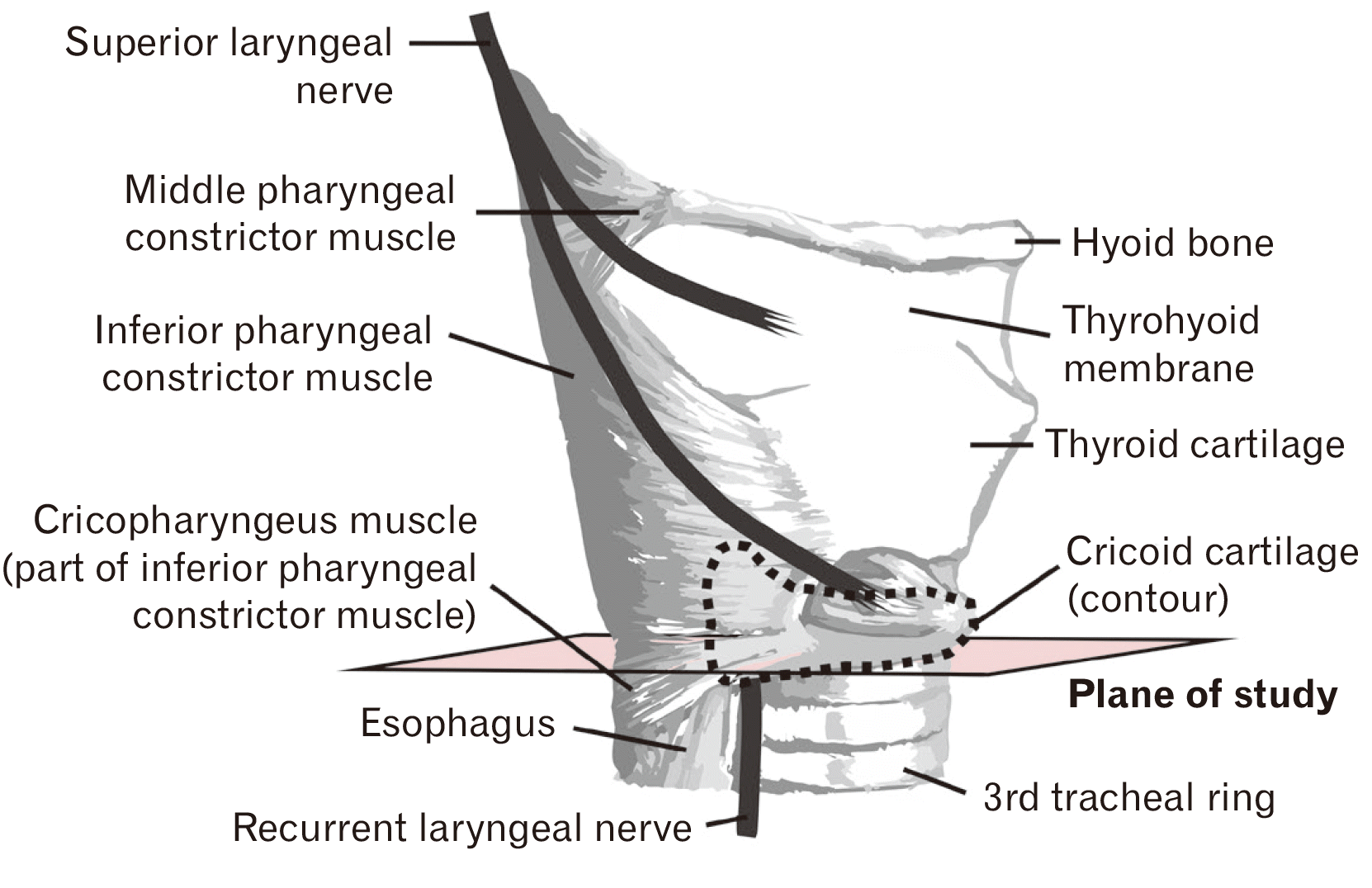
Fig. 2
Recurrent laryngeal nerve in human fetuses. (A, B) Photomacrography of the vagal detachment of the right and left recurrent laryngeal nerves. Red, aorta and its branches. Turquoise, phrenic nerve. Blue, pulmonary trunk. Purple, ductus arteriosus. Yellow, vagus nerve. Green, recurrent laryngeal nerve. Tr, trachea; Lg, right lung; Hr, heart. Scale bar=1 cm.
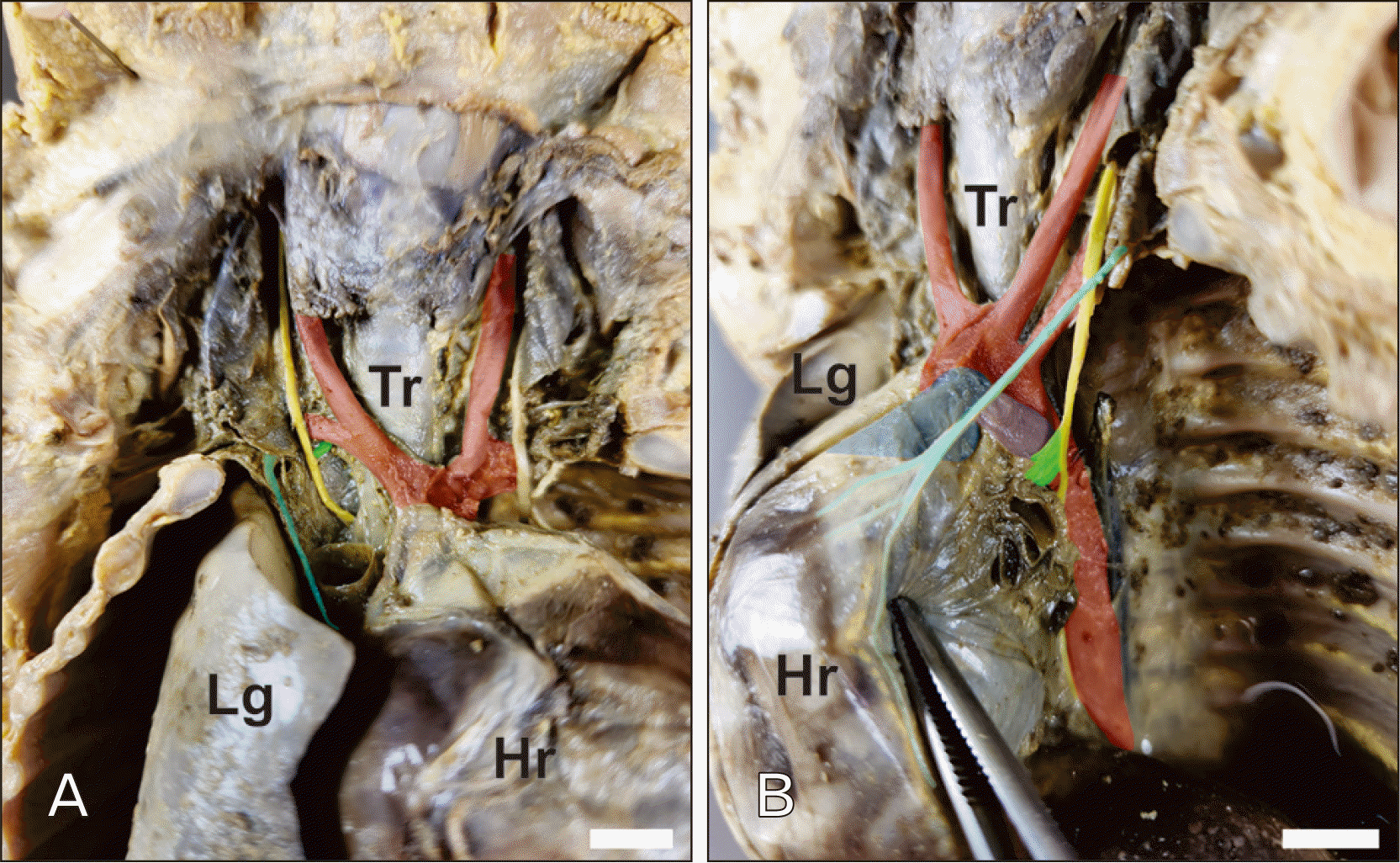
Fig. 3
Histological preparation and cross-sectional morphometry of the recurrent laryngeal nerve. (A) Photomicrography of the right recurrent laryngeal nerve (RLN) superficially to the cricoid cartilage (CC) and deep to the cricopharyngeus muscle (CPM). Scale bar=100 µm. (B) Inset of the RLN showing the analyzed area of histology slides for epineurium (black line), and perineural space (yellow shadow). Note the presence of two vasa nervorum within the epineurium and nerve axons enveloped by the perineural epithelium. Scale bar=50 µm. (C) Cross-sectional area of the epineurium (I) and perineural space (II) from the right (R) and left (L) recurrent laryngeal nerves. R and L scores from the same subjects are connected in the plot. ns, non significant P>0.05.
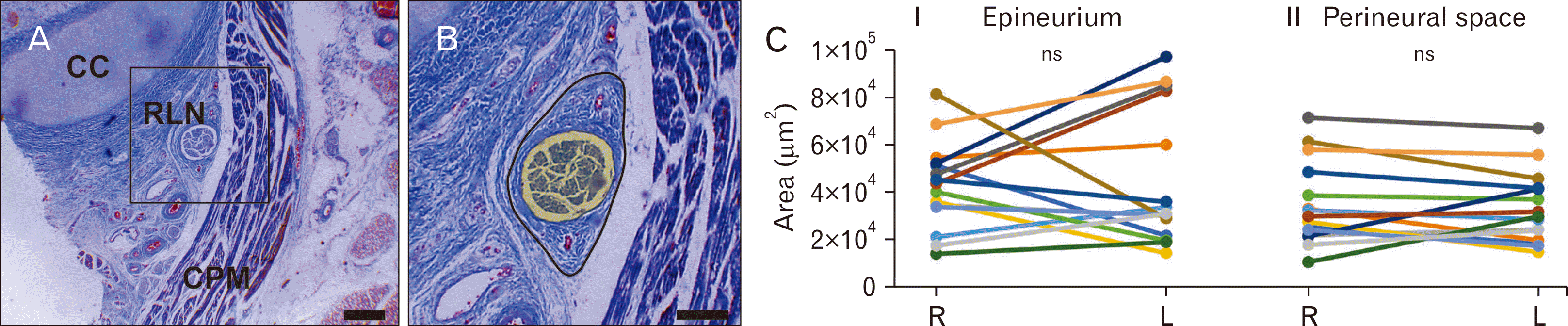
Fig. 4
Development of the epineurium and perineural space from 30 to 40 weeks. (A) Scatter plot showing the relationship between gestational age and cross-section morphometry of the perineurium in right (I) and left (II) recurrent laryngeal nerves. (B) Scatter plot showing the relationship between gestational age and cross-section morphometry of the perineural space in right (I) and left (II) recurrent laryngeal nerves. Linear regression equation, correlation coefficient and probability values are represented by y, R, and P within the plots.
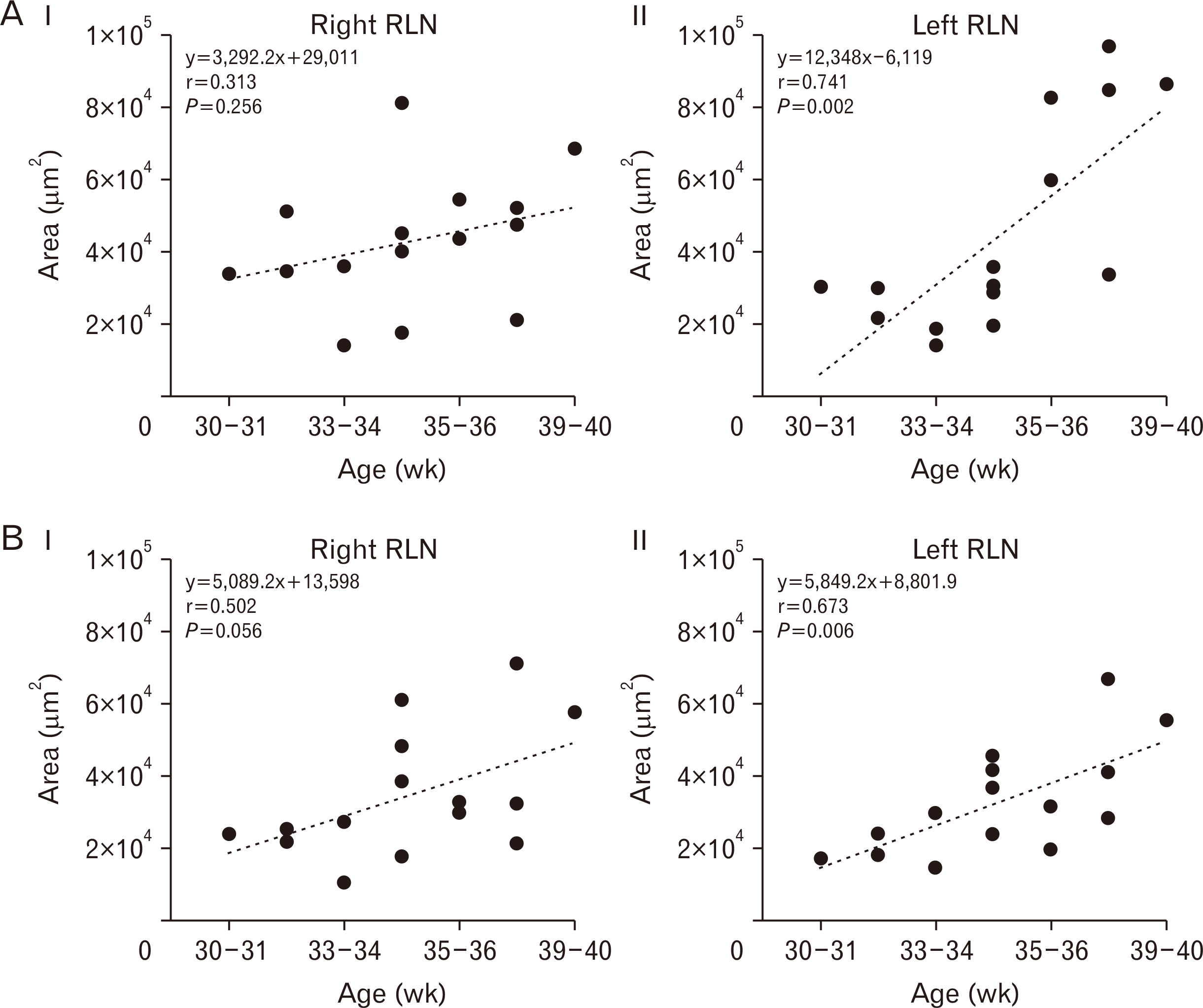
Table 1
Fetal inventory and cross-sectional area of the recurrent laryngeal nerve
Table 2
Fetal inventory and perineural cross-sectional space morphometry of the recurrent laryngeal nerve