Abstract
The endocrinology of type 2 diabetes (T2D) and its predisposing factors have been studied extensively while its skeletal effects have received negligible research despite this being a global disease. The cellular and molecular association between proximal humeral fractures and T2D has not been fully elucidated. We aimed to study bone cell quantities and immunolabel osteogenic and antiosteogenic cytokines. The study used 12-week-old rats (23 males) consisting of 8 Sprague Dawley (SD) and 15 Zucker Diabetic Sprague Dawley (ZDSD). Weekly mass measurements were taken while fasting blood glucose levels were recorded every 2 weeks with oral glucose tolerance tests conducted once every 4 weeks. Upon termination at the age of 28 weeks, humeri were fixed in 10% buffered formalin, prior to decalcification in ethylenediaminetetraacetic acid. The bone samples were then processed in ascending grades of alcohol using an automatic processor before embedding in paraffin wax. Sections were cut at 5 µm thickness in a series for Haematoxylin and Eosin stain, and immunohistochemistry was performed with the anti-tartrate-resistant acid phosphatase (TRAP), anti-alkaline phosphatase (ALP), anti-bone morphogenetic protein 3 (BMP3), anti-transforming growth factor beta 1 (TGFβ1), anti-aged glycation end product (AGE) antibodies in the sequence. ZDSD rats had more adipocytes, BMP3 and AGEs expression with higher numbers of TRAP positive osteocytes and fewer ALP cells although no differences were found in TGFβ1 immunopositivity. We also found that T2D increases the number of AGEs immuno-positive cells, as well as its extracellular expression, thus providing a conducive environment for the interaction of the osteogenic cytokine and its antagonist to suppress osteoblastogenesis. ZDSD groups had higher adipocyte numbers therefore increased marrow adiposity in T2D.
The endocrinology of type 2 diabetes (T2D) and its predisposing factors have been studied extensively [1] while its skeletal effects have received negligible research [2]. Fractures in T2D are exacerbated by hyperglycemia and disease progression [3, 4]. Proximal humerus fractures (PHF) account for 4% to 10% of all fractures, constituting the 2nd most common fractures of the upper arm [5, 6], and the 3rd commonest fracture site after the proximal femur and distal radius [7]. A study on the humerus of female T2D Goto-Kakizaki rats demonstrated that fracture risk in T2D varies with specific sub regions in bones [8].
The cellular and molecular association between PHF and T2D has not been fully elucidated. However, previous studies suggest that T2D induces osteopenia [5] that compromises bone turnover [9, 10], as well as an imbalance between pro and anti-osteogenic cytokines [11, 12]. Reduced bone turnover is directly linked to altered bone cell numbers and their function [12-14].
Tartrate-resistant acid phosphatase (TRAP) as contained in osteocytes, participates in osteolysis in response to pathologic conditions such as T2D [15, 16]. Additionally, TRAP positive osteocytes, also found in elevated levels in T2D, attract osteoclast precursors to their vicinity, accelerating bone matrix resorption and increasing bone fragility [17, 18]. Osteoblasts secrete osteoid and mineralize the organic matrix under the influence of ALP [19]. Studies on the femur of the Zucker Diabetic Fatty rat found impaired osteoblast differentiation, reduced osteoblast numbers and less matrix mineralization in diabetes [8, 20, 21].
The extracellular bone matrix cytokine transforming growth factor beta 1 (TGFβ1) [22, 23] is responsible for regulating differentiation, proliferation, and migration of osteoprogenitor cells [24, 25]. In contrast, bone morphogenetic protein 3 (BMP3) is antagonistic to the TGFβ1 functions [23]. In addition, T2D facilitates accumulation of extracellular aged glycation end products (AGEs) that adversely crosslink bone matrix collagen, compromising skeletal integrity [26]. This occurs by inhibiting osteoblast differentiation and proliferation and induction of osteoblast apoptosis while increasing osteoclastic and osteocytic bone catabolism [27-30]. Relatively recent studies show that increased skeletal fragility in T2D is exacerbated by bone marrow adiposity due to the differentiation of skeletal stem cells into adipogenic cell lines instead of osteogenic cell lines [31, 32].
We aimed to compare bone cell quantities and immunolabel osteogenic and antiosteogenic cytokines in the Zucker Diabetic Sprague Dawley (ZDSD) and SD rat humerus. In this study, we will start by assessing bone samples for adipocyte accumulation. We will then quantify the bone turnover cells namely, osteocytes and osteoblasts using TRAP and ALP as cell markers respectively. Expression of the pro-osteogenic cytokine TGFβ1 [33], and the anti-osteogenic cytokine BMP3 [23], will be investigated, as they are reported to be altered in T2D [11]. The extent of glycation end-product accumulation will be assessed by quantifying AGEs immunopositive bone cells together with its extracellular expression.
Approval for use of animals was received from the Animal Ethics Screening Committee of the University of the Witwatersrand (clearance number: 2015/07/28C). The study used 23 male rats consisting of 8 SD and 15 ZDSD. The SD rats, which served as controls were sourced from the Central Animal Services (CAS) University of the Witwatersrand. The SD rats were used as the non-diabetic controls because they are the parental strains of the ZDSD rats [26]. All animals were housed one per cage, maintained under pathogen free conditions, in a temperature-controlled environment of 23°C±2°C under a 12-hour light and dark cycle.
The ZDSD rats were further divided into moderate diabetes (ZDSD-MOD) (n=9) and severe diabetes (ZDSD-SVD) groups (n=6). The diabetic groups were defined based on oral glucose tolerance test (OGTT) as follows: (1) Moderately diabetic (ZDSD-MOD) animals had >8.0 mmol/L fasting blood glucose (FBG) prior to glucose load and >12.5 mmol/L 2 hours after glucose loading, and more than 50% increase in area under the curve (AUC) after OGTT. (2) Severely diabetic animals (ZDSD-SVD) had >11.2 mmol/L FBG prior to glucose load and >23.0 mmol/L 2 hours after glucose loading, and more than 200% increase in AUC.
All animals were fed ad libitum a standard Purina 5008 chow (Lab diet) and given water, ad libitum. At 15 weeks, the ZDSD rats were changed into a high fat diet (5SCA; Test diet), where 47.7% of the kilocalories is derived from fat. These animals were switched back to the standard Purina 5008 chow after 6 weeks. The high fat diet was used to synchronize the onset of hyperglycemia in the ZDSD rats [26].
Animals were fasted for 12 hours overnight prior to blood glucose measurements. A glucometer (Performa Accutrend; Roche Diagnostics) was used to measure FBG by lancing the tail vein, every 2 weeks.
Oral glucose tolerance tests (OGTTs) were performed monthly following a 12 hours fast. Fasting glucose levels were recorded at time zero (T0) before receiving an oral glucose load (2 g/kg). Glucose levels were measured from blood collected from the tail vein at 15, 30, 60, and 120 minutes intervals after the glucose load.
At the age of 28 weeks, the animals were injected with a lethal intraperitoneal dose of phenobarbital. Humeri were removed, and muscles cleaned out. Individual bones were then stored in 10% buffered formalin for fixation until further processing.
Bone samples were fixed in 10% buffered formalin for a minimum of 14 days, then decalcified in 20% w/v ethylenediaminetetraacetic acid (pH 7.4) for 10 days in an incubator at 45°C with regular gentle shaking. The bones were then rinsed, and their proximal epiphysis severed about 3 mm below the intertubercular groove, then immersed in 10% buffered formalin for 24 hours before processing for histology. An automatic processor (Citadel 2000; Thermo Fischer Scientific) was used to take the samples through ascending grades of ethanol prior to embedding in paraffin wax. During embedding, bone samples were orientated longitudinally to minimize differences in the sectioning angle. Bone sections were cut at 5 μm thickness using a microtome (Leica RM2125 RTS; Leica Biosystems) and mounted on silane-coated glass slides. Three sections per slide were mounted. Section sequences were mounted in order for specific stains, namely; Hematoxylin and Eosin (H&E) to assess humerus head and morphology, and to quantify adipocyte numbers; Immunolocalization of TRAP, ALP, BMP3, TGFβ1, and AGEs for the same region.
Sections were dewaxed in xylene for 10 minutes and hydrated by passing through 3 changes (1 minute each) of absolute alcohol followed by 90% and 70% alcohol (1 minute each) and eventually into running tap water for 1 minute. The sections were then stained for 10 minutes in haematoxylin, differentiated in periodic acid for 10 seconds, rinsed in tap water for a minute, blued in Scott’s tap water for 2 minutes, and rinsed again in tap water. The cytoplasm was stained with eosin by immersing the sections in 70% alcohol followed by eosin for 2 minutes. The sections were then dehydrated in increasing concentrations of alcohol before mounting in entellen.
Immunohistochemistry was conducted using various antibodies (Table 1). All antibodies were sourced from Abcam. Sections were incubated at 40°C for 30 minutes, deparaffinized and rehydrated in a series of descending grades of alcohols, then immersed in a citrate buffer (pH=6) and placed in a water bath set at 60°C for 24 hours. This was to facilitate antigen retrieval. After 24 hours sections were cooled at room temperature for 20 minutes then rinsed 3×5 minutes times in phosphatebuffered saline (PBS; pH=7.4). Endogenous peroxidase was blocked with 1% hydrogen peroxide (H2O2) and washed in PBS 3×5 minutes. Sections were transferred to a perspex plate and incubated with primary antibodies at appropriate dilutions (Table 1) and placed at 4°C overnight. After 24 hours, sections were acclimatized for 20 minutes until room temperature, before washing in PBS (pH=7.4), 3×5 minutes. Sections were soaked in biotinylated secondary antibody (Biotinylated Goat Anti-Rabbit IgG (H+L) (ab64256) for 10 minutes, again washed in PBS (pH=7.4) 3×5 minutes. They were incubated further in streptavidin HRP for 10 minutes (ab64269) to reduce background immunopositivity and enhance antibody binding. PBS (pH=7.4) rinses were performed (3×5 minutes). Sections were then incubated with 3-3’ di-aminobenzidine working solution while monitoring color development under a light microscope. Once optimal immunopositivity was achieved, sections were, rinsed in running tap water for 5 minutes, then dehydrated through graded alcohol, cleared in xylene and mounted on silane-coated slides and entellen.
A light microscope (Zeiss Axioscope 2 plus) fitted with an Axiocam HRC digital camera connected to a PC was used to take photomicrographs of the stained slides. Anatomical landmarks were determined for the humerus head, using the H&E stained slides, to create consistency in quantification and immunopositivity analysis. Nonadjacent fields were selected, two above and two below the epiphyseal growth plate (Fig. 1). Photomicrographs were imported into Fiji Image-J software (National Institute of Health USA) for further analysis. TRAP positive osteocytes [15, 34], ALP positive osteoblasts [35, 36], AGEs positive bone cells and adipocytes were identified as specified in previous literature [31]. Cells were counted manually. Immunolocalization for anti-AGEs antibody, anti-BMP3 antibody, and anti-TGFβ1 antibody was performed. These were graded as no stain, mild, moderate, and intense immunopositivity (Fig. 1).
The data were managed in Microsoft Excel Office 365 (Microsoft Corporation) and all data were analyzed using SPSS® version 28 (IBM Co.). The data were tested for normality using the Shapiro–Wilks test, the data was found to be normally distributed, and a one-way ANOVA with LSD post-hoc test was applied for multiple group comparisons. Ordinal data for immunopositivity intensity were assigned grading scales of no stain (0), mild (1), moderate (2) and intense (3). Significance level was set at P<0.05.
Animals in all the study groups showed a steady body mass gain up to week-17. By week-18, the ZDSD-MOD (530.31±22.50 g) and ZDSD-SVD (519.67±10.45 g) had a lower body mass compared to SD controls (540±12.18 g) (Fig. 2A). As such, significant differences were observed between SD and both ZDSD-MOD and ZDSD-SVD (P<0.001) from week 18 until termination. At 28 weeks the SDs (610.75± 20.66 g) had gained 70.63 g compared to both ZDSD-MOD (521.45±11.23 g) and ZDSD-SVD (490±20.15 g) that had lost 8.86 g and 29.47 g respectively (Fig. 2A).
The controls exhibited lower values compared to diabetics, with SDs (4.99±0.31 mmol/L) recording lower glucose levels compared to ZDSD-MOD (7.11±0.13 mmol/L) and ZDSD-SVD (13.38±1.61 mmol/L) (P=0.019, P<0.001 respectively) (Fig. 2B).
The SD (4.70 mmol/L) controls exhibit lower glucose values at T0 compared to ZDSD-MOD (8.25 mmol min/dl) and ZDSD-SVD (11.20 mmol/L) (P=0.014, P<0.001 respectively) (Fig. 2C). This significant trend was maintained 2hrs post administration of the glucose bolus, with SD controls (6.53 mmol/L) showing lower values than ZDSD-MOD (12.97 mmol/L) together with the ZDSD-SVD (23.27 mmol/L) (P<0.001 for both comparisons) (Fig. 2C). The ZDSD-MOD (1,314±136.5 mmol min/dl) and ZDSD-SVD (2,434±320.60 mmol min/dl) showed an increase (71.2% and 217% respectively) in mean AUC when compared to SD (767.50±29.64 mmol min/dl) controls (Fig. 2C).
Most of the ZDSD-MOD and ZDSD-SVD animals exhibited mild immunopositivity. The remaining ZDSD-MOD and 1 ZDSD-SVD had moderate immunopositivity. The SD controls exhibited mostly mild, moderate (in equal proportions) with 1 animal showing intense immunopositivity (Table 2). This observation between the SD controls, ZDSD-MOD and ZDSD-SVD ZDSDs was statistically similar (P=0.206, P=0.103 respectively) (Table 2, Fig. 6).
All ZDSD-SVD rats exhibit intense BMP3 immunopositivity with majority of ZDSD-MOD exhibiting mild and moderate immunopositivity (Table 2). Most SD controls had mild immunopositivity with only 1 animal exhibiting no immunopositivity. Significant differences were observed between the SD controls and both groups of ZDSDs (P=0.002, P<0.001 respectively) (Table 2, Fig. 6).
Quantification of adipocytes revealed increased adiposity in the ZDSDs similar to other rat [37], and human studies [38]. TRAP and ALP immunopositive cells quantities showed that T2D promotes osteolysis and inhibits osteoblastogenesis in ZDSD rats. To understand the role of cytokines in the ZDSD rat skeletal elements, we assessed the expression of one osteogenic cytokine (TGFβ1) as well as an antagonist (BMP3). The ZDSDs had more BMP3 expression and relatively less TGFβ1. This shows that T2D affects bone cells though suppression TGFβ1 expression while its antagonist (BMP3) is elevated.
Multiple studies have elucidated how T2D contributes to increased bone marrow adiposity. This adipogenic influence of T2D on mesenchymal stem cell enhances the development of adipocytogenic cell lines while suppressing osteogenic cell lines [31, 37, 39, 40]. These marrow adipocytes secrete the hormone adiponectin that is anti-osteoblastogenic, pro-osteoclastogenic and promotes osteoblast apoptosis [29, 40]. This could partially explain the susceptibility of bones to fracture in diabetes [31, 32]. In the present study, the ZDSDs exhibited higher adipocyte numbers compared to age matched controls. Our observation implies that T2D contribute to increased bone marrow adiposity [37] in the head of the humerus.
To evaluate the proposition that osteocytes are active [15, 17, 41], and not dormant as previously thought [27], these cells were immuno-labeled using the anti-TRAP antibody. TRAP is typically used as an osteoclast marker; however, recent studies have shown that osteocytes express the bone matrix catabolic enzyme TRAP as they engage in osteolysis or peri lacuna-canaliculi remodeling [15, 41]. This causes direct resorption and deposition of the bone matrix in osteocytes’ immediate environment in response to pathologic conditions like T2D [15, 16, 34, 41]. In the present study, significantly more TRAP immunopositive osteocytes were observed in the ZDSDs and their age matched controls in alignment with previous research [4]. These TRAP immunopositive cells trigger processes that attract osteoclast precursors to their vicinity, leading to increased bone matrix resorption [17, 18], thus contributing to osteolysis.
Osteoblastic ALP promotes osteoid formation and bone mineralization [19]. It is used as a bone turnover marker in the diagnosis of bone disease [19, 42, 43]. Studies on animal models of T2D have reported impaired osteoblast differentiation, reduced osteoblast numbers and poor matrix mineralization in T2D. This is thought to lead to increased bone fragility [8, 20, 21]. In the present study, ZDSDs had fewer ALP positive osteoblasts. This finding supports the proposition that T2D inhibits osteoblastogenesis. This may further explain the known bone fragility in T2D.
Type 2 diabetes increases the expression of AGEs both intracellularly and in the extracellular matrix [44]. This AGEs accumulation increases with age and diabetes progression [44, 45]. It adversely affects intracellular signaling molecules that encode for osteoblast, osteoclast, and osteocyte functions [4, 26, 45, 46]. A possibility exists that this high AGEs accumulation could interfere with the TGFβ1 receptors, preventing entry of the extracelullar TGFβ1 into the cells, thereby interfering with the osteoblastogenic role of this cytokine.
The present study refers to the secreted cytokine rather than intracellular TGFβ1. Therefore, the extracellular TGFβ1 amounts may not be directly engaged in the cellular activities such as the well-known transcription for osteoprogenitor cell differentiation and function [22]. A possibility exists that the AGEs accumulation may block the interaction of TGFβ1 with cellular components required for osteoblastogeneis gene encoding [45].
In our study, ZDSDs exhibited either moderate or intense BMP3 immunopositivity, with no immunopositivity in the controls. This observation suggests that T2D reduces the expression of BMP3, and possibly suppresses osteoblast function [23, 47]. In support of this proposition, previous research reports increased osteoblast activity in BMP3 null mice [47]. Notably, intense immunopositivity was observed in the overtly diabetic group, possibly suggesting that BMP-3 over expression may be associated with excessive hyperglycemia.
In conclusion, we found that diabetes affected osteoblastogenesis as measured by ALP positive cells and bone marrow adipocytes. TRAP positive osteocytes numbers were increased in the presence of T2D, suggesting an increased osteolysis. The ZDSD groups had less osteogenic cytokine (TGFβ1) expression, with more of the antagonist (BMP3) expression. We also found that T2D increases the number of AGEs immuno-positive cells, as well as its extracellular expression, thus providing a conducive environment for the interaction of the osteogenic cytokine and its antagonist to suppress osteoblastogenesis. ZDSD groups had higher adipocyte numbers therefore increased marrow adiposity in T2D. We conclude that T2D adversely affects bone turnover cells, leading to reduced osteoblastogenesis and increased osteolysis the ZDSD rat humerus.
Acknowledgements
We are grateful to Ms Hasiena Ali for technical assistance and to the staff of the CAS at the University of the Witwatersrand for assistance with animal handling and welfare.
The views and opinions expressed are those of the authors and do not necessarily represent the official views of the SA MRC.
Notes
References
1. Oh YS. 2015; Mechanistic insights into pancreatic beta-cell mass regulation by glucose and free fatty acids. Anat Cell Biol. 48:16–24. DOI: 10.5115/acb.2015.48.1.16. PMID: 25806118. PMCID: PMC4371177.


2. Del Prato S. 2009; Role of glucotoxicity and lipotoxicity in the pathophysiology of type 2 diabetes mellitus and emerging treatment strategies. Diabet Med. 26:1185–92. DOI: 10.1111/j.1464-5491.2009.02847.x. PMID: 20002468.


3. Devlin MJ, Van Vliet M, Motyl K, Karim L, Brooks DJ, Louis L, Conlon C, Rosen CJ, Bouxsein ML. 2014; Early-onset type 2 diabetes impairs skeletal acquisition in the male TALLYHO/JngJ mouse. Endocrinology. 155:3806–16. DOI: 10.1210/en.2014-1041. PMID: 25051433. PMCID: PMC4164927.


4. Picke AK, Campbell G, Napoli N, Hofbauer LC, Rauner M. 2019; Update on the impact of type 2 diabetes mellitus on bone metabolism and material properties. Endocr Connect. 8:R55–70. DOI: 10.1530/EC-18-0456. PMID: 30772871. PMCID: PMC6391903.


5. Chu SP, Kelsey JL, Keegan TH, Sternfeld B, Prill M, Quesenberry CP, Sidney S. 2004; Risk factors for proximal humerus fracture. Am J Epidemiol. 160:360–7. DOI: 10.1093/aje/kwh224. PMID: 15286021.
6. Iglesias-Rodríguez S, Domínguez-Prado DM, García-Reza A, Fernández-Fernández D, Pérez-Alfonso E, García-Piñeiro J, Castro-Menéndez M. 2021; Epidemiology of proximal humerus fractures. J Orthop Surg Res. 16:402. DOI: 10.1186/s13018-021-02551-x. PMID: 34158100. PMCID: PMC8220679. PMID: 9ce944b37e9c43278bfa1ec533b19e2d.


7. Chen Y, Lin C, Huang X, Lin F, Luo X. 2021; Comparison of treatment results between surgical and conservative treatment of distal radius fractures in adults: a meta-analysis of randomized controlled trials. Acta Orthop Traumatol Turc. 55:118–26. DOI: 10.5152/j.aott.2021.20168. PMID: 33847573. PMID: 5f316bb719fb44ba9683210cd39f3591.
8. Ahmad T, Ohlsson C, Sääf M, Ostenson CG, Kreicbergs A. 2003; Skeletal changes in type-2 diabetic Goto-Kakizaki rats. J Endocrinol. 178:111–6. DOI: 10.1677/joe.0.1780111. PMID: 12844342.


9. Martinez-Huedo MA, Jiménez-García R, Mora-Zamorano E, Hernández-Barrera V, Villanueva-Martinez M, Lopez-de-Andres A. 2017; Trends in incidence of proximal humerus fractures, surgical procedures and outcomes among elderly hospitalized patients with and without type 2 diabetes in Spain (2001-2013). BMC Musculoskelet Disord. 18:522. DOI: 10.1186/s12891-017-1892-7. PMID: 29228945. PMCID: PMC5725839. PMID: 890a8e34a20b4b02974c2dce7ed2ea1b.


10. Schousboe JT, Morin SN, Kline GA, Lix LM, Leslie WD. 2022; Differential risk of fracture attributable to type 2 diabetes mellitus according to skeletal site. Bone. 154:116220. DOI: 10.1016/j.bone.2021.116220. PMID: 34571204.


11. Sassi F, Buondonno I, Luppi C, Spertino E, Stratta E, Di Stefano M, Ravazzoli M, Isaia G, Trento M, Passera P, Porta M, Isaia GC, D'Amelio P. 2018; Type 2 diabetes affects bone cells precursors and bone turnover. BMC Endocr Disord. 18:55. DOI: 10.1186/s12902-018-0283-x. PMID: 30089481. PMCID: PMC6083573. PMID: cf8510fa38ed40d4935939e3bf05647c.


12. Seibel MJ. 2005; Biochemical markers of bone turnover: part I: biochemistry and variability. Clin Biochem Rev. 26:97–122. DOI: 10.1007/978-1-59745-459-9_5. PMID: 16648882. PMCID: PMC1320175.
13. Feng X, McDonald JM. 2011; Disorders of bone remodeling. Annu Rev Pathol. 6:121–45. DOI: 10.1146/annurev-pathol-011110-130203. PMID: 20936937. PMCID: PMC3571087.


14. Neer RM, Arnaud CD, Zanchetta JR, Prince R, Gaich GA, Reginster JY, Hodsman AB, Eriksen EF, Ish-Shalom S, Genant HK, Wang O, Mitlak BH. 2001; Effect of parathyroid hormone (1-34) on fractures and bone mineral density in postmenopausal women with osteoporosis. N Engl J Med. 344:1434–41. DOI: 10.1056/NEJM200105103441904. PMID: 11346808.


15. Dole NS, Mazur CM, Acevedo C, Lopez JP, Monteiro DA, Fowler TW, Gludovatz B, Walsh F, Regan JN, Messina S, Evans DS, Lang TF, Zhang B, Ritchie RO, Mohammad KS, Alliston T. 2017; Osteocyte-intrinsic TGF-β signaling regulates bone quality through perilacunar/canalicular remodeling. Cell Rep. 21:2585–96. DOI: 10.1016/j.celrep.2017.10.115. PMID: 29186693. PMCID: PMC6014615. PMID: 0fca8e4f2a204101aeb0cc7062150b19.


16. Qing H, Ardeshirpour L, Pajevic PD, Dusevich V, Jähn K, Kato S, Wysolmerski J, Bonewald LF. 2012; Demonstration of osteocytic perilacunar/canalicular remodeling in mice during lactation. J Bone Miner Res. 27:1018–29. DOI: 10.1002/jbmr.1567. PMID: 22308018. PMCID: PMC3770147.


17. Bellido T. 2014; Osteocyte-driven bone remodeling. Calcif Tissue Int. 94:25–34. DOI: 10.1007/s00223-013-9774-y. PMID: 24002178. PMCID: PMC3947228.


18. Burger EH, Klein-Nulend J. 1999; Mechanotransduction in bone--role of the lacuno-canalicular network. FASEB J. 13 Suppl:S101–12. DOI: 10.1096/fasebj.13.9001.s101. PMID: 10352151.


19. Bhattarai T, Bhattacharya K, Chaudhuri P, Sengupta P. 2014; Correlation of common biochemical markers for bone turnover, serum calcium, and alkaline phosphatase in post-menopausal women. Malays J Med Sci. 21:58–61. PMID: 24639613. PMCID: PMC3952340.
20. Blakytny R, Spraul M, Jude EB. 2011; Review: the diabetic bone: a cellular and molecular perspective. Int J Low Extrem Wounds. 10:16–32. DOI: 10.1177/1534734611400256. PMID: 21444607.


21. Hamann C, Goettsch C, Mettelsiefen J, Henkenjohann V, Rauner M, Hempel U, Bernhardt R, Fratzl-Zelman N, Roschger P, Rammelt S, Günther KP, Hofbauer LC. 2011; Delayed bone regeneration and low bone mass in a rat model of insulin-resistant type 2 diabetes mellitus is due to impaired osteoblast function. Am J Physiol Endocrinol Metab. 301:E1220–8. DOI: 10.1152/ajpendo.00378.2011. PMID: 21900121.


22. Ehnert S, Baur J, Schmitt A, Neumaier M, Lucke M, Dooley S, Vester H, Wildemann B, Stöckle U, Nussler AK. 2010; TGF-β1 as possible link between loss of bone mineral density and chronic inflammation. PLoS One. 5:e14073. DOI: 10.1371/journal.pone.0014073. PMID: 21124921. PMCID: PMC2989906. PMID: af8b5d35ea694327bcd1ef5dec8edee9.


23. Wu M, Chen G, Li YP. 2016; TGF-β and BMP signaling in osteoblast, skeletal development, and bone formation, homeostasis and disease. Bone Res. 4:16009. DOI: 10.1038/boneres.2016.9. PMID: 27563484. PMCID: PMC4985055.


24. Janssens K, ten Dijke P, Janssens S, Van Hul W. 2005; Transforming growth factor-beta1 to the bone. Endocr Rev. 26:743–74. DOI: 10.1210/er.2004-0001. PMID: 15901668.
25. Jian H, Shen X, Liu I, Semenov M, He X, Wang XF. 2006; Smad3-dependent nuclear translocation of beta-catenin is required for TGF-beta1-induced proliferation of bone marrow-derived adult human mesenchymal stem cells. Genes Dev. 20:666–74. DOI: 10.1101/gad.1388806. PMID: 16543220. PMCID: PMC1413283.
26. Creecy A, Uppuganti S, Merkel AR, O'Neal D, Makowski AJ, Granke M, Voziyan P, Nyman JS. 2016; Changes in the fracture resistance of bone with the progression of type 2 diabetes in the ZDSD rat. Calcif Tissue Int. 99:289–301. DOI: 10.1007/s00223-016-0149-z. PMID: 27209312. PMCID: PMC4961536.


27. Plotkin LI, Essex AL, Davis HM. 2019; RAGE signaling in skeletal biology. Curr Osteoporos Rep. 17:16–25. DOI: 10.1007/s11914-019-00499-w. PMID: 30685821. PMCID: PMC6447316.


28. Sanguineti R, Puddu A, Mach F, Montecucco F, Viviani GL. 2014; Advanced glycation end products play adverse proinflammatory activities in osteoporosis. Mediators Inflamm. 2014:975872. DOI: 10.1155/2014/975872. PMID: 24771986. PMCID: PMC3977495. PMID: 18b5ff2636874b30bd7496a39332e851.


29. Singh L, Tyagi S, Myers D, Duque G. 2018; Good, bad, or ugly: the biological roles of bone marrow fat. Curr Osteoporos Rep. 16:130–7. DOI: 10.1007/s11914-018-0427-y. PMID: 29476394.


30. Yamamoto M, Sugimoto T. 2016; Advanced glycation end products, diabetes, and bone strength. Curr Osteoporos Rep. 14:320–6. DOI: 10.1007/s11914-016-0332-1. PMID: 27704396. PMCID: PMC5104767.


31. Ferland-McCollough D, Maselli D, Spinetti G, Sambataro M, Sullivan N, Blom A, Madeddu P. 2018; MCP-1 feedback loop between adipocytes and mesenchymal stromal cells causes fat accumulation and contributes to hematopoietic stem cell rarefaction in the bone marrow of patients with diabetes. Diabetes. 67:1380–94. DOI: 10.2337/db18-0044. PMID: 29703845.


32. Gillet C, Dalla Valle A, Gaspard N, Spruyt D, Vertongen P, Lechanteur J, Rigutto S, Dragan ER, Heuschling A, Gangji V, Rasschaert J. 2017; Osteonecrosis of the femoral head: lipotoxicity exacerbation in MSC and modifications of the bone marrow fluid. Endocrinology. 158:490–502. DOI: 10.1210/en.2016-1687. PMID: 28359085.
33. Yang Y, Zhang N, Lan F, Van Crombruggen K, Fang L, Hu G, Hong S, Bachert C. 2014; Transforming growth factor-beta 1 pathways in inflammatory airway diseases. Allergy. 69:699–707. DOI: 10.1111/all.12403. PMID: 24750111.


34. Nakano Y, Toyosawa S, Takano Y. 2004; Eccentric localization of osteocytes expressing enzymatic activities, protein, and mRNA signals for type 5 tartrate-resistant acid phosphatase (TRAP). J Histochem Cytochem. 52:1475–82. DOI: 10.1369/jhc.4A6378.2004. PMID: 15505342. PMCID: PMC3957824.


35. Hawthorne AC, Xavier SP, Okamoto R, Salvador SL, Antunes AA, Salata LA. 2013; Immunohistochemical, tomographic, and histological study on onlay bone graft remodeling. Part III: allografts. Clin Oral Implants Res. 24:1164–72. DOI: 10.1111/j.1600-0501.2012.02528.x. PMID: 22775764.


36. Miao D, Scutt A. 2002; Histochemical localization of alkaline phosphatase activity in decalcified bone and cartilage. J Histochem Cytochem. 50:333–40. DOI: 10.1177/002215540205000305. PMID: 11850436.


37. Kim TY, Schafer AL. 2016; Diabetes and bone marrow adiposity. Curr Osteoporos Rep. 14:337–44. DOI: 10.1007/s11914-016-0336-x. PMID: 27714580. PMCID: PMC5126977.


38. Fairfield H, Falank C, Harris E, Demambro V, McDonald M, Pettitt JA, Mohanty ST, Croucher P, Kramer I, Kneissel M, Rosen CJ, Reagan MR. 2018; The skeletal cell-derived molecule sclerostin drives bone marrow adipogenesis. J Cell Physiol. 233:1156–67. DOI: 10.1002/jcp.25976. PMID: 28460416. PMCID: PMC5664178.


39. Piccinin MA, Khan ZA. 2014; Pathophysiological role of enhanced bone marrow adipogenesis in diabetic complications. Adipocyte. 3:263–72. DOI: 10.4161/adip.32215. PMID: 26317050. PMCID: PMC4550679.


40. Rharass T, Lucas S. 2018; Mechanisms in endocrinology: bone marrow adiposity and bone, a bad romance? Eur J Endocrinol. 179:R165–82. DOI: 10.1530/EJE-18-0182. PMID: 30299886.


41. Kaya S, Basta-Pljakic J, Seref-Ferlengez Z, Majeska RJ, Cardoso L, Bromage TG, Zhang Q, Flach CR, Mendelsohn R, Yakar S, Fritton SP, Schaffler MB. 2017; Lactation-induced changes in the volume of osteocyte lacunar-canalicular space alter mechanical properties in cortical bone tissue. J Bone Miner Res. 32:688–97. DOI: 10.1002/jbmr.3044. PMID: 27859586. PMCID: PMC5395324.


42. Čepelak I, Čvorišćec D. 2009; Biochemical markers of bone remodeling - review. Biochem Med. 19:17–35. DOI: 10.11613/BM.2009.003.


43. Florencio-Silva R, Sasso GR, Sasso-Cerri E, Simões MJ, Cerri PS. 2015; Biology of bone tissue: structure, function, and factors that influence bone cells. Biomed Res Int. 2015:421746. DOI: 10.1155/2015/421746. PMID: 26247020. PMCID: PMC4515490.


44. Goldin A, Beckman JA, Schmidt AM, Creager MA. 2006; Advanced glycation end products: sparking the development of diabetic vascular injury. Circulation. 114:597–605. DOI: 10.1161/CIRCULATIONAHA.106.621854. PMID: 16894049.
45. Singh VP, Bali A, Singh N, Jaggi AS. 2014; Advanced glycation end products and diabetic complications. Korean J Physiol Pharmacol. 18:1–14. DOI: 10.4196/kjpp.2014.18.1.1. PMID: 24634591. PMCID: PMC3951818.


46. Kislinger T, Fu C, Huber B, Qu W, Taguchi A, Du Yan S, Hofmann M, Yan SF, Pischetsrieder M, Stern D, Schmidt AM. 1999; N(epsilon)-(carboxymethyl)lysine adducts of proteins are ligands for receptor for advanced glycation end products that activate cell signaling pathways and modulate gene expression. J Biol Chem. 274:31740–9. DOI: 10.1074/jbc.274.44.31740. PMID: 10531386.
47. Gazzerro E, Canalis E. 2006; Bone morphogenetic proteins and their antagonists. Rev Endocr Metab Disord. 7:51–65. DOI: 10.1007/s11154-006-9000-6. PMID: 17029022.


Fig. 1
Humerus head regions of interest. The squares represent nonadjacent areas from which photomicrographs were taken.
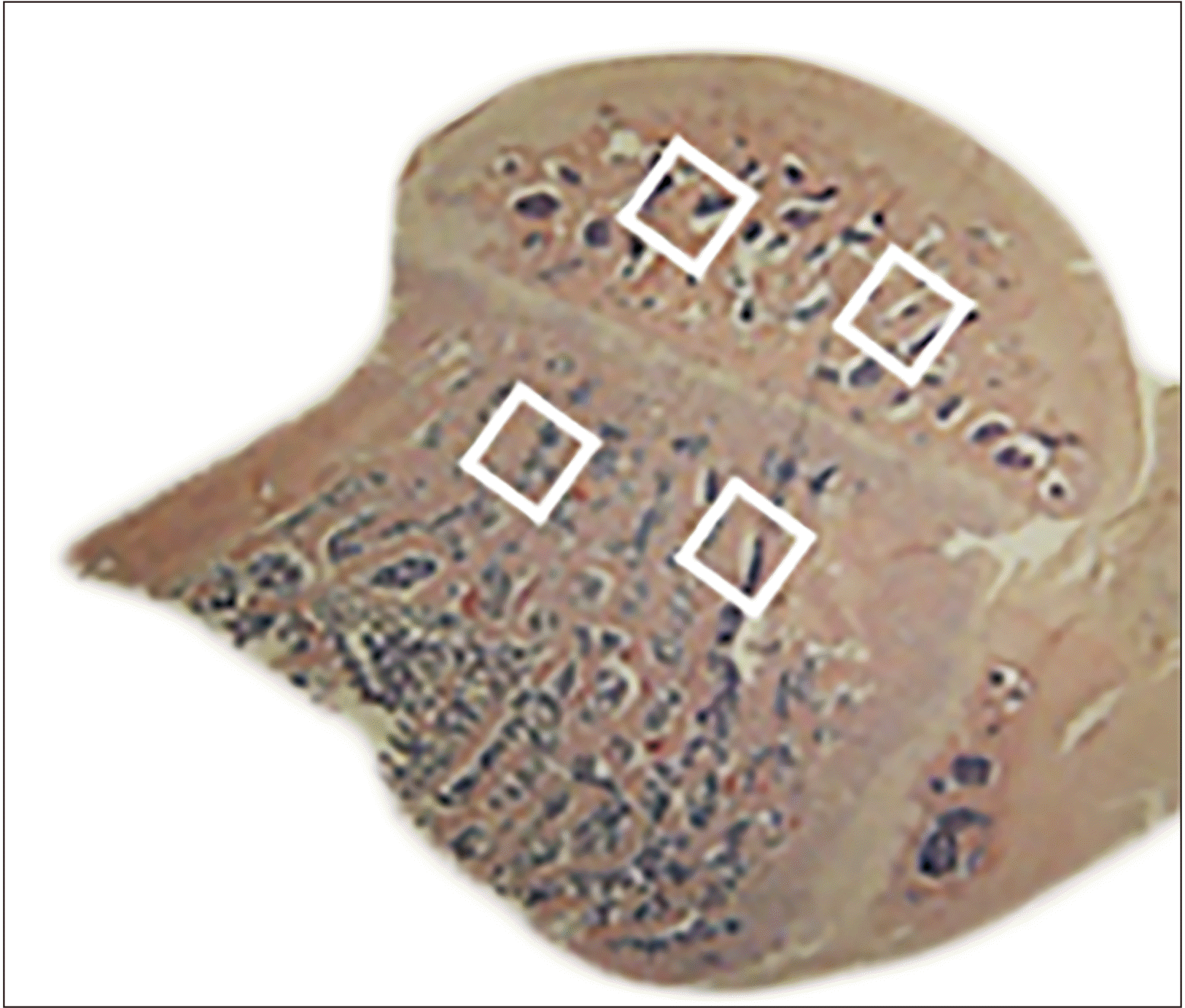
Fig. 2
Body mass and glucose handling. (A) A gradual reduction in mass is exhibited from week 18 by ZDSD-MOD and ZDSD-SVD when compared to SD (*P<0.001 for both). (B) Fasting blood glucose SD vs. ZDSD-MOD and ZDSD-SVD (P=0.019, P<0.001 respectively). (C) OGTT and AUC shows inefficient glucose disposal by the ZDSD-SVD and ZDSD-MOD compared to the SD (P≤0.001, P=0.014 respectively). The ZDSD-SVD had the largest mean AUC (P<0.001), followed by the ZDSD-MOD when compared with the SD controls (P=0.014). ZDSD-MOD and ZDSD-SVD; ZDSD rats moderately diabetic and severely diabetic, respectively. Errors bars represent standard deviation. SD, Sprague Dawley; ZDSD-MOD, Zucker Diabetic Sprague Dawley rats were further divided into moderate diabetes; ZDSD-SVD, ZDSD rats were further divided into severe diabetes; OGTT, oral glucose tolerance test; AUC, area under the curve.
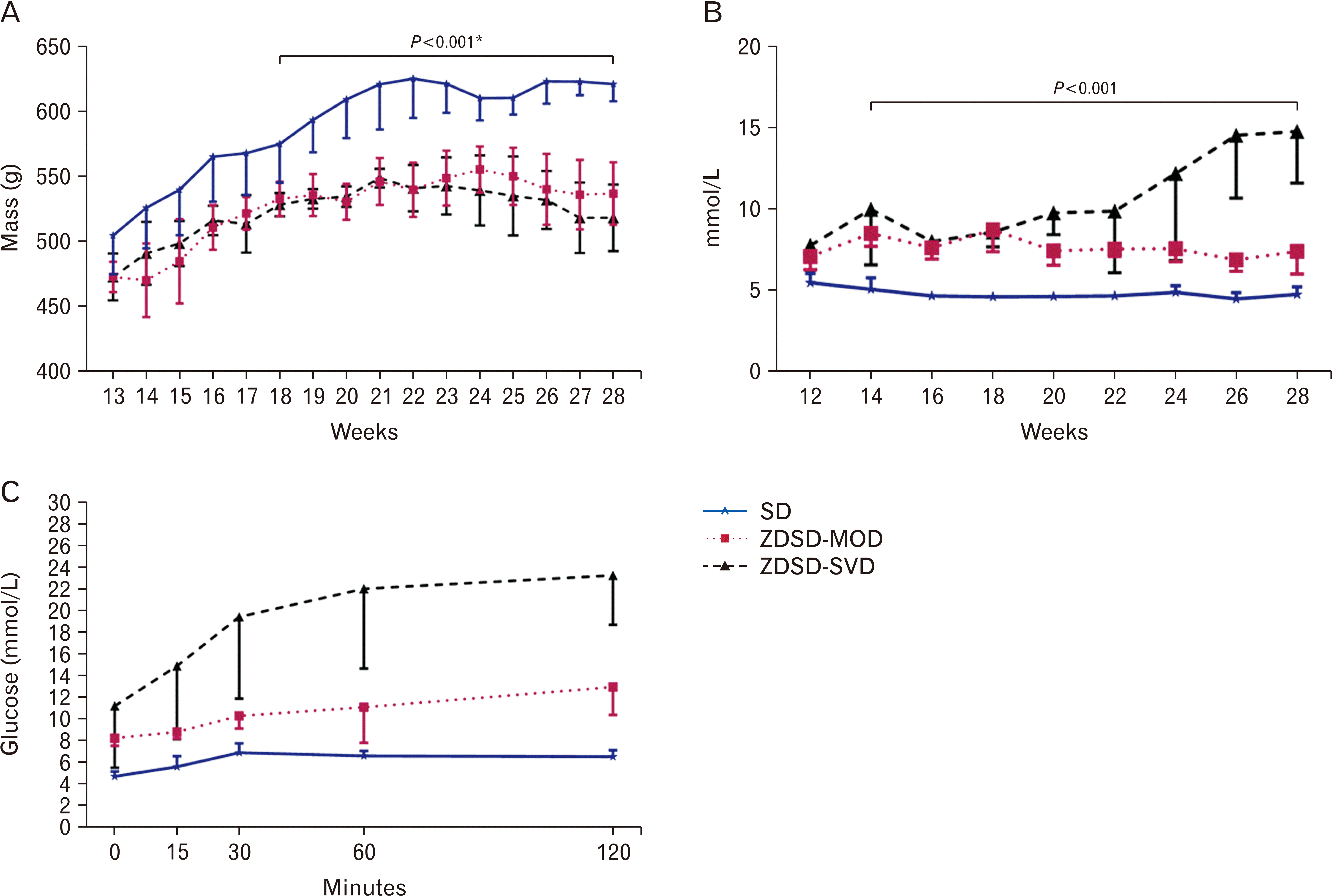
Fig. 3
General histology and relative adipocyte infiltration in the humerus head. Adipocytes are the large round cells with a clear cytoplasm as depicted by the arrow in the insert. (A) SD, no obvious adipocytes. (B) ZDSD-MOD, some adipocytes are visible. ZDSD-SVD, numerous adipocytes throughout the bone marrow. Scale bar represents 40 μm and applies to A–C. SD, Sprague Dawley; ZDSD-MOD, Zucker Diabetic Sprague Dawley rats that had moderate diabetes; ZDSD-SVD, Zucker Diabetic Sprague Dawley rats that had severe diabetes.

Fig. 4
Cell counts. (A) Number of adipocytes in the humerus head. P<0.001 when comparing SD with ZDSD-MOD and ZDSD-SVD, respectively. (B) TRAP immunopositive cells. P=0.001 and P<0.001 when comparing SD with ZDSD-MOD and ZDSD-SVD respectively. (C) ALP immunopositive cells. P<0.001 when comparing SD against ZDSD-MOD and ZDSD-SVD, respectively. (D) AGEs immunopositive cells. P<0.001 when comparing ZDSD-MOD and ZDSD-SVD, respectively. Box and Whisker plots (A–D). The line inside the box represents the mean, whereas the bottom and top lines of the box show the 25th and 75th percentiles, respectively. The whiskers below and above the box show the minimum and maximum values, respectively. SD, Sprague Dawley; ZDSD-MOD, Zucker Diabetic Sprague Dawley rats that had moderate diabetes; ZDSD-SVD, Zucker Diabetic Sprague Dawley rats that had severe diabetes; TRAP, tartrate-resistant acid phosphatase; ALP, alkaline phosphatase; AGE, aged glycation end products.
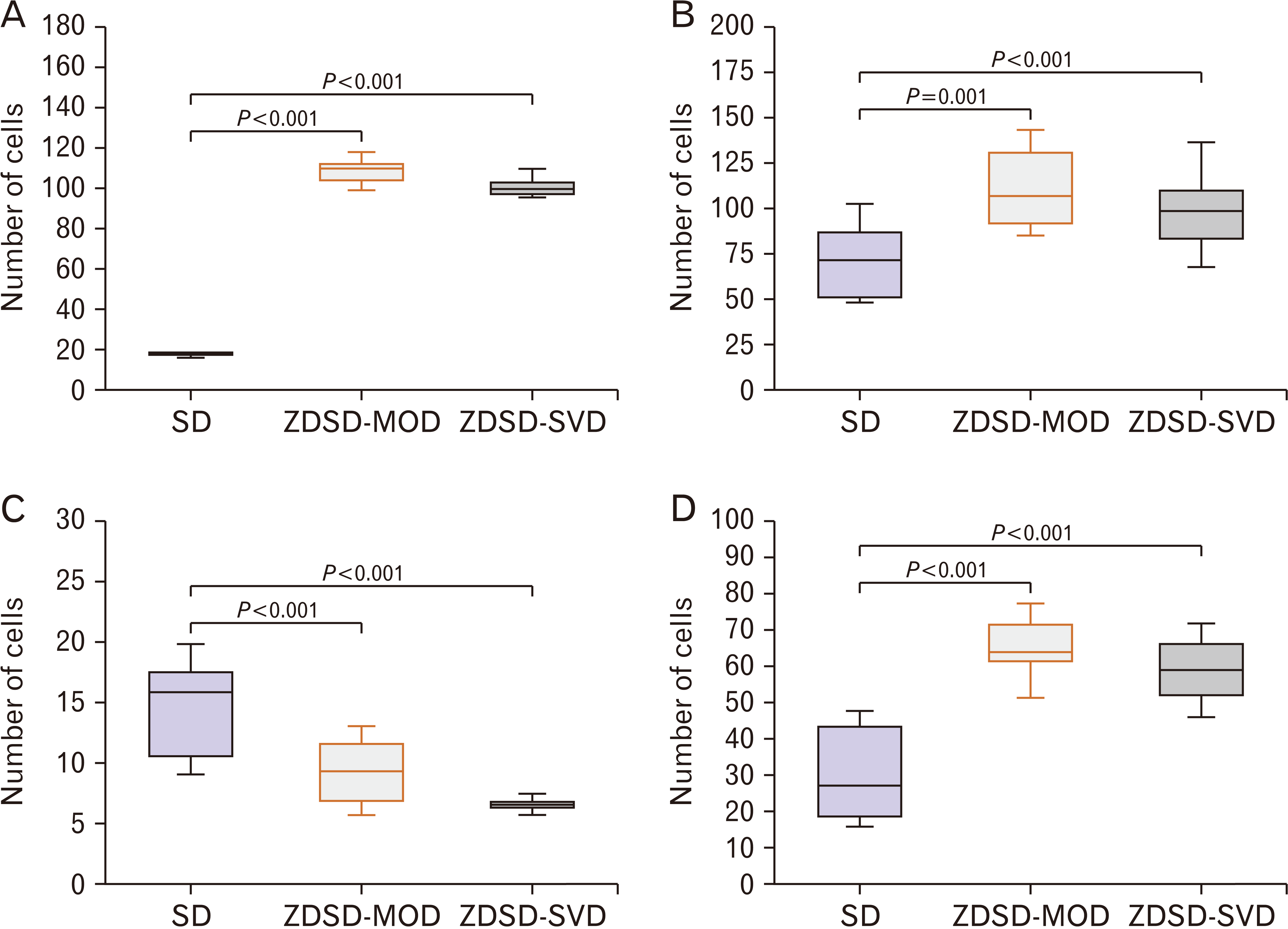
Fig. 5
ALP Immunopositive osteoblasts. TRAP immunopositive cells are withing trabeculae (A–C). The osteoblasts lining bone marrow spaces are visible (D–F) as well as the AGEs immunopositive cells (G–I). The arrows show examples of the respective cell types. Scale bar represents 40 μm and applies all photomicrographs. SD, Sprague Dawley; ZDSD-MOD, Zucker Diabetic Sprague Dawley rats that had moderate diabetes; ZDSD-SVD, Zucker Diabetic Sprague Dawley rats that had severe diabetes; ALP, alkaline phosphatase; TRAP, tartrate-resistant acid phosphatase; AGE, aged glycation end products.
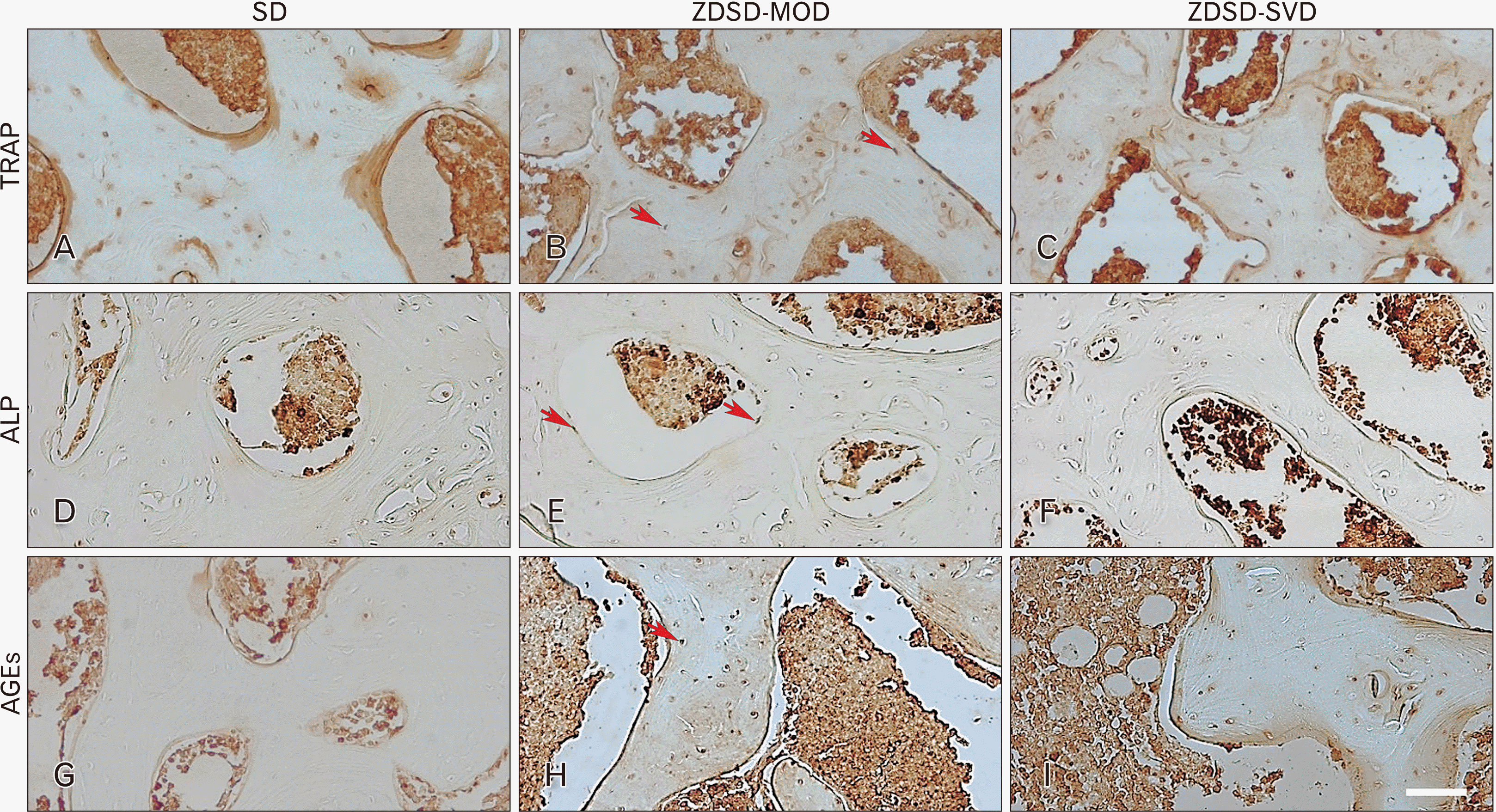
Fig. 6
TGFβ1 and BMP3 Immunopositivity grading. Scale bar represents 100 µm and applies to all photomicrographs. TGFβ1, transforming growth factor beta 1; BMP3, bone morphogenetic protein 3.
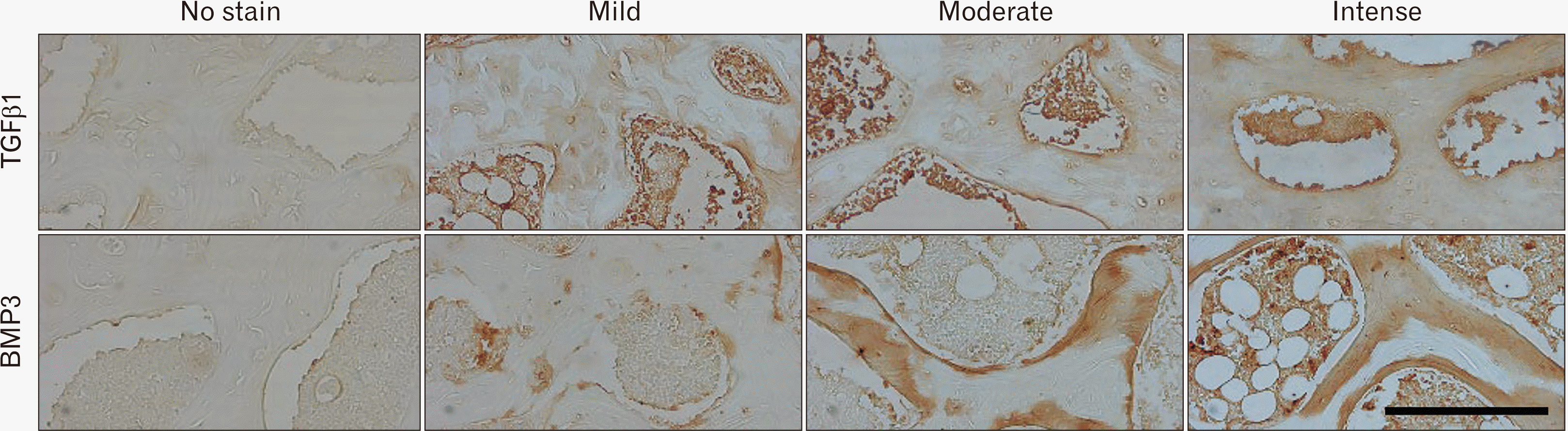
Table 1
List of antibodies and dilution
Table 2
TGFβ1 and BMP3 immunopositivity
Group | N | No stain | Mild | Moderate | Intense | P-value |
---|---|---|---|---|---|---|
TGFβ1 | ||||||
SD | 7 | 0 (0) | 3 (42.9) | 3 (42.9) | 1 (14.3) | |
ZDSD-MOD | 9 | 0 (0) | 6 (66.7) | 3 (33.3) | 0 (0) | 0.203 |
ZDSD-SVD | 6 | 0 (0) | 5 (83.3) | 1 (16.7) | 0 (0) | 0.103a) |
BMP3 | ||||||
SD | 7 | 1 (14.3) | 6 (85.7) | 0 (0) | 0 (0) | |
ZDSD-MOD | 9 | 0 (0) | 4 (44.4) | 5 (55.6) | 0 (0) | 0.002 |
ZDSD-SVD | 6 | 0 (0) | 0 (0) | 0 (0) | 6 (100) | <0.001a) |
Values are presented as number only or number (%). TGFβ1, transforming growth factor beta 1; BMP3, bone morphogenetic protein 3; SD, Sprague Dawley; ZDSD-MOD, Zucker Diabetic Sprague Dawley rats that had moderate diabetes; ZDSD-SVD, Zucker Diabetic Sprague Dawley rats that had severe diabetes. a)Comparison of SD with ZDSD-SVD.