Abstract
Ultrasound is a widely used diagnostic medical imaging modality, and its therapeutic potential has recently been reported. This study examined whether ultrasound irradiation causes nanobubble destruction and identified the most effective frequency and duration of ultrasound for doxorubicin delivery in rat liver under ultrasound-targeted nanobubble destruc-tion. Rats underwent an intravenous nanobubble injection via the caudal vein. An ultrasound probe was applied to the rat liver. The rats were divided into different groups based on the frequency. Fluorescence images were acquired using an IVIS® system. Laboratory tests were performed to determine the serum levels of AST (aspartate aminotransferase), ALT (alanine aminotransferase), total bilirubin, and creatinine. Under ultrasound irradiation, more nanobubbles were destroyed, and the doxorubicin uptake was increased in the rat liver (P < 0.05). The 5 MHz group showed an increased fluorescence efficiency in the liver (P < 0.05). Based on the duration of ultrasound irradiation, the 5, 10, and 13 MHz groups showed an increased fluorescence efficiency in the liver compared to the control group (P < 0.05). There was no significant difference between the 15- and 30-minute groups. The other organs showed no significant differences compared to the control group. The groups to which ultrasound was applied showed increased serum AST and ALT levels, but the effects did not last for 30 min. Ultrasound-targeted nanobubble destruction was effective for doxorubicin delivery in the rat liver. The most effective ultrasound frequency and irradiation duration were 5 MHz and 15 min, respectively.
An ultrasonic device is a non-invasive, affordable real-time imaging device, which does not involve radiation exposure; therefore, it is used as an important diagnostic tool in clinical practices.(1) In addition to the traditional ultrasonic diagnostic technique that uses the difference in reflected ultrasound between tissues to obtain information, an ultrasound contrast agent, which injects microbubbles and highlights the acoustic impedance differences between lesions and their surrounding areas, has been commercialized, and it has improved the diagnostic value of the ultrasonic device.(2) Recently, studies have been conducted where ultrasonic devices are used not only for diagnosis, but also as a tool to treat diseases. There have been many attempts to improve the local drug effect by administering microbubbles, which are used as an ultrasound contrast agent, providing ultrasound irradiation, and destroying them at a certain location. A previous study described an experiment in which only microbubbles were administered and irradiated with ultrasound, and the effects of ultrasound on the microbubbles were examined.(3) Alternatively, there are methods that show the treatment effect by administering microbubbles attached to drugs or genes, followed by ultrasound irradiation. This improves the absorption rate of the medication or genes in the target area.(4-7) The aforementioned methods use a phenomenon where physical damage in the capillary wall is caused by microbubbles, which resonate in accordance with the amplitude of ultrasound waves, show repeated fluctuations in size, and are destroyed in the end. This method is based on the theory that microbubbles are destroyed under ultrasound irradiation and split into smaller parts. This makes it easier for drugs or genes to permeate the tissue, because the enhanced permeability and retention effect in cancerous tumors increase the size of the blood vessel walls inside the tumors allowing faster tumor growth than in the normal tissues.(8)
Lately, studies on appropriate ultrasound contrast agents are being conducted, including attachment and administering of drugs in nanometer-sized nanoparticles, destroying them under ultrasound irradiation, and verifying their effect on drug delivery.(9) However, additional studies on a substance appropriate for drug delivery based on ultrasound-targeted microbubble destruction and the frequency of the ultrasound waves, as well as their applications, are needed.
In this study, laboratory white rats were administered nanobubbles developed from nanoparticles containing doxorubicin (DOX), a chemotherapy drug used in liver cancer treatment, and ultrasound irradiation was performed on the liver. Then, we examined whether the nanobubbles were destroyed inside the body. We aimed to identify the ultrasound frequency and ultrasound irradiation duration appropriate for drug delivery, and its effect on liver parenchyma.
Hydrophobically modified glycol chitosan (HGC) was developed through chemical bonding between glycol chitosan (Sigma Chemical Co. St. Louis, MO), whose molecular weight is 250 kDa, and 5β-cholanic acid (Sigma Chemical Co.), followed by melting in distilled water to transform it into the water phase glycol chitosan nanoparticles (GCNPs). It was mixed with doxorubicin-HCL (DOX) (Sigma Chemical Co.) with the same water phase at the ratio of 1:3, and DOX-GCNP was manufactured using the water-in-water emulsification method. Then, we added perfluoro-pentane (PFP; Apollo Scientific Ltd. Manchester, UK), which was in the oil phase, to the water phase DOX-GCNP and synthesized a substance with echogenic properties through the oil-in-water emulsification method (DOX-NB) (Figs. 1 and 2).(10)
Sprague-Dawley white rats weighing 200-250 g were used for this experiment. They were acclimatized to the laboratory environment for one week before the experiment.
They were given general anesthesia by isoflurane inhalation (Ifran Liq.®, HanaPharm, Gyeonggi-do, Korea) and laid on the operating table. The experimental conditions were maintained at a temperature of 37℃ (Ani1400T-TC, LMSKorea, Gyeonggi-do, Korea). The limbs of the rats were tied and held in position while we used the ultrasound device (F37®, Hitachi Aloka medical, Tokyo, Japan) and located their liver. The ultrasonic probe was fastened to a fixed device so that it would not move during the experiment (Fig. 3).
The prepared 3% 1 mL DOX-NB was administered intravenously through the caudal vein of the rat, and ultrasound irradiation was performed depending on the experimental group.
All the experiments were conducted under the approval and supervision of the Animal Ethics Committee of Saint Paul’s Hospital of Catholic University of Korea (SPH-20151217-01) and complied with the animal experiment guidelines.
(1) Control group (n = 6, no ultrasound group): DOX-NB was administered without ultrasound irradiation.
(2) 5 MHz group (n = 6, 5 MHz group): DOX-NB was administered with an ultrasound frequency of 5 MHz, MI 1.2 was irradiated for 15 min.
(3) 10 MHz group (n = 6, 10 MHz group): DOX-NB was administered with an ultrasound frequency of 10 MHz, MI 1.2 was irradiated for 15 min.
(4) 13 MHz group (n = 6, 13 MHz group): DOX-NB was administered with an ultrasound frequency of 13 MHz, MI 1.2 was irradiated for 15 min.
(1) Control group (n = 6, no ultrasound group): DOX-NB was administered without ultrasound irradiation.
(2) 15 minute group (n = 6, 15 min group): DOX-NB was administered with an ultrasound frequency of 5 MHz, MI 1.2 was irradiated for 15 min.
(3) 30 minute group (n = 6, 30 min group): DOX-NB was administered with an ultrasound frequency of 5 MHz, MI 1.2 was irradiated for 30 min.
DOX itself is a florescent chemotherapy drug, and the distribution of the drug was estimated by measuring its ex vivo fluorescence, and it has been used in various experiments.(11,12) In this experiment, DOX florescence was measured to identify DOX distribution in each organ.
The rats were sacrificed after the experiment, and their liver, kidney, heart, and lung were recovered and fixed in formalin. Using an optical in vivo imaging system (IVIS Lumina WRMS, PerkinElmer, Waltham, MA), florescence imaging was recorded at absorption and emission wavelengths of 470 and 540 nm, respectively, to identify ex vivo DOX distribution in each organ. In the recorded images, the range of interest (ROI) was measured using the living image software of IVIS® spectrum.
The laboratory white rats were sacrificed after the experiment, and their blood samples were collected from the inferior vena cava and centrifuged. Aspartate aminotransferase (AST), alanine aminotransferase (ALT), albumin, total bilirubin, and creatinine (Cr) levels in the blood were measured to test the liver and kidney functions.
All 50 rats distributed randomly into five groups survived during the experiment period. The average weight was 232.5 ± 15.1 g, and the temperature during the surgery was maintained at 36-37℃.
The liver parenchyma, inferior vena cava, hepatic vein, and aorta were seen in the ultrasonic images taken during the experiments. After administration of nanoparticles, although no contrast enhancement was observed in the liver parenchyma, slight contrast enhancement in the inferior vena cava of the 10 and 13 MHz groups was observed (Fig. 4).
DOX-NB was intravenously administered to the rats, and florescence efficiency was measured in the liver, kidney, lung, and heart for each ultrasound frequency range. The average florescence efficiency detected in the liver in all experimental groups was 47.00 × 109, which was far higher than the other organs (P < 0.001). For other organs, the average in all experimental groups was in the order of the kidney (7.72 × 109), lung (2.89 × 109), and heart (2.67 × 109) (Fig. 5).
Among the experimental groups, no statistically significant difference in the kidney, lung, and heart was observed. However, the florescence efficiency detected in the liver in each group showed statistically significant differences (P = 0.005). The florescence efficiency of the 5 MHz group was significantly higher than that of the other groups (P < 0.05). The 10 and 13 MHz groups showed a higher florescence efficiency than the control group. The florescence efficiency tended to decrease, but did not show a statistical significance (Table 1).
The fluorescence efficiency in each organ after intravenous administration of DOX-NB to the rats showed no difference in florescence in the organs except the liver, in accordance with the ultrasound irradiation duration. The liver showed statistically significant differences in the detected florescence efficiency in accordance with the ultrasound irradiation duration (P = 0.027). While there was no difference between the 15 and 30 minute groups, the florescence efficiency in the ultrasound irradiation groups was significantly higher than that in the control group (P < 0.05; Table 2). In other organs except the liver, ultrasound irradiation groups did not show significant differences compared to the control group, nor was there any significant difference in florescence efficiency in accordance with ultrasound irradiation duration.
The control group and 5 MHz group, which showed a significant difference, were compared. The results showed that while there was no difference between the two groups in the kidney, heart, and lung, florescence efficiency in the liver in the 5 MHz group increased significantly (P = 0.013; Fig. 6).
Fluorescence efficiency in the liver of the control group was 73.4%, which increased by approximately 10% to 83% in the 5 MHz group (Fig. 7).
In a biochemical test performed with the serum, the ALT of the groups for each ultrasound irradiation frequency showed statistical significance (P = 0.014), and the ALT of the ultrasound irradiation groups had risen significantly compared to that in the control group (P < 0.05). AST, albumin, and creatinine did not show statistical differences (Table 3).
AST levels showed statistical significance among the experimental groups (P = 0.018). Although there was a statistically significant increase in AST between the control and 15 min group, no significant difference between the 15 and 30 min groups were observed (P < 0.05). ALT showed a similar pattern to AST, but there was no statistical significance. Albumin and creatinine did not show any statistical difference (P = 0.080; Table 4).
Since its first application in the 1940s in clinical practices, ultrasonic methods for disease diagnosis and treatment have made considerable progress and expanded its application areas.(13) Recently, many studies are being conducted for the use of ultrasound in disease treatment. Notably, high-intensity focused ultrasound (HIFU), which locally irradiates highly intense ultrasound and generates heat for treatment, has been studied. Another study focused on the use of low-intensity ultrasound for producing local cavitation.(3) Other studies were conducted for the treatment of malignant as well as benign diseases via thrombolysis, gene therapy, transfection, and drug delivery using microbubbles and local ultrasound irradiation to the target lesion.(14-16)
The traditional chemotherapy used for liver cancer has limitations because the dose required to induce the death of cancer cells is too high, which results in systemic side effects. Alternatively, treatment techniques such as transarterial chemoembolization (TACE) for liver cancer or radiofrequency ablation (RFA) are used; however, these techniques are also invasive.(17-19) To overcome these limitations, several researchers conducted studies in which DNA or chemotherapy drugs were attached to microbubbles, and the applicability of microbubbles to liver cancer therapy was examined. Ultrasound irradiation of the microbubbles increased the local concentration of the substances in the liver.(20,21) Their study showed that the microbubbles in the bloodstream were destroyed by local ultrasound irradiation, which increased the concentration of encapsulated substances in the target organ. In addition, the destruction of microbubbles changed the permeability of capillaries, which raised the blood vessel and tissue permeability of the substances intended to be delivered.(15)
Microbubbles have limitations in that the onset of action decreases as they tend to be dispersed naturally inside. In addition, their concentration can be easily reduced by a natural clean-up process inside the body. Moreover, their capillary permeability is limited as their diameter is 1 µm or greater, which is bigger than the 380-780 nm hole in the capillary wall, formed inside the tumor tissue during angiogenesis.(8,22-24) The nanobubbles used in this experiment (796.6 ± 142.2 nm in average size) could overcome this limitation as they were synthesized with nanoparticles and PFP, whose average size was 287 ± 25 nm, and maintained at a size of 1 µm or less for 30 min at 37℃, the assumed temperature of the living body.(10) In addition, we attached the chemotherapy medication paclitaxel to nanoparticles—the nanobubble precursors—for the ex vivo experiment. The results showed that it was easier to attach the drug to the nanoparticles in this experiment than other nanoparticles, and the natural loss of the chemotherapy drug from the nanoparticles was low. In addition, nanobubbles have the advantage that they can lower systemic toxicity in the in vivo experiment while showing the same therapeutic effect as other nanoparticles.(25) In this ex vivo experiment, more DOX was absorbed into the cell nucleus through nanobubbles attached to DOX than through ordinary nanobubbles. Consequently, the DOX concentration in the target organ increased, confirming that systemic toxicity could be lowered. Thus, this study showed the efficacy of drug delivery using nano-bubbles.(10)
The results of this experiment showed that florescence efficiency in the liver was 5 to 10 times higher than that in other organs, and the DOX distribution in the liver was high. The mononuclear phagocytic system (MPS), by which the florescence efficiency in liver is more abundantly distributed than in other organs, is known to contribute to the faster absorption of nanobubbles.(20,26) Accordingly, we confirmed that ultrasound irradiation to the liver destroyed nanobubbles intensely distributed by the MPS and enhanced the local drug effect. Nanobubbles are expected to play a useful role in research on liver cancer or diseases in the future.
The frequency range mostly used in ex vivo and in vivo ultrasound-targeted microbubble destruction (UTMD) experiments is 0.4 to 1.75 MHz of the research device. It is different from the frequency range of the ultrasound device widely used in clinical practices.(5,6,14,15,21,27,28) Although there is no detailed analysis report on bubble destruction effect being dependent on the frequency range, we could have a sufficient destruction effect in the range of 3 to 10 MHz, which is mostly used in clinical practices.(29) Unlike microbubble research, nanobubble experiments mostly use the frequency range of 5 to 7 MHz, which is used in clinical practices.(22,30) The results of this study showed that florescence was detected more in the 5 MHz group than in the other groups. As it was much easier to destroy nanobubbles in the liver at 5 MHz frequency than at 10 and 13 MHz, it was confirmed that 5 MHz was the adequate frequency for ultrasound irradiation.
Among all organs, the percentage of detected florescence efficiency in the liver increased 10% in the 5 MHz group than in the control group, which demonstrated that the nanobubble destruction effect was maximized in the liver after irradiation at 5 MHz frequency. Thus, it was observed that ultrasound irradiation was effective in delivering an intended drug to the liver.
The ex vivo nanobubble experiment results of glycol chitosan used in this experiment showed that its size was maintained at 1 µm or less for no longer than 30 min at 37℃. Therefore, rats were divided into 15 and 30 min groups based on the ultrasound irradiation duration for the experiment. It was found that it was not necessary to extend the ultrasound irradiation duration more than 15 min, as the detected florescence efficiency was high in the 15 min group, while not much difference was observed in the 30 min group. In a similar microbubble experiment, Wang et al. injected Evans blue dye and microbubbles in a liver cancer rat model, irradiated ultrasound, and examined the amount of absorbed dye with the passage of time. They reported that the amount reached the highest at 10 min, and extending the time did not make a difference.(6)
To assess the short-term effect of nanobubbles on the liver and kidney functions, we used blood samples extracted after ultrasound irradiation and tested the serum levels of enzyme and creatinine. No change was observed in the creatinine level when compared to the control group. The effect of nanobubbles on the kidney function was not very significant. The liver enzyme level was consistent with the rise in florescence efficiency. It reached its peak at 5 MHz, and no further increase was observed when the frequency increased. For ultrasound irradiation duration, however, the liver enzyme level tended to fall in the 30 min group compared to the 15 min group. This was consistent with the findings of Yang et al. that although the liver enzyme level rose temporarily in rats after microbubbles were injected owing to the increase in their cell membrane permeability in vascular endothelial cells, the level dropped to the normal level with time.(5)
In conclusion, it was observed that after administration of nanobubbles attached with the chemotherapy drug DOX to white rats and ultrasound irradiation to the liver, florescence efficiency increased in the liver, demonstrating that the drug was locally delivered. The appropriate ultrasound frequency to destroy nanobubbles was considered 5 MHz, while ultrasound irradiation duration of 15 min showed the largest effect. In the liver function test, no continuous drop in liver enzyme level was found. However, these results were observed in a normal liver; therefore, a study on liver cancer animal models would be needed to apply these results for the treatment of cancer in the future.
REFERENCES
1. Juffermans LJ, Dijkmans PA, Musters RJ, van Wamel A, Bouakaz A, Ten Cate FJ, et al. 2004; Local drug and gene delivery through microbubbles and ultrasound: a safe and efficient alternative for viral vectors? Neth Heart J. 12:394–9.
2. Xu J, Wu Y, Dong F. 2007; Clinical value of contrast-enhanced ultrasound in differentiating benign and malignant focal liver lesions. J Huazhong Univ Sci Technolog Med Sci. 27:703–5. DOI: 10.1007/s11596-007-0622-z. PMID: 18231748.


3. Yumita N, Nishigaki R, Umemura S. 2000; Sonodynamically induced antitumor effect of Photofrin II on colon 26 carcinoma. J Cancer Res Clin Oncol. 126:601–6. DOI: 10.1007/PL00008471. PMID: 11043398.


4. Liu P, Wang X, Zhou S, Hua X, Liu Z, Gao Y. 2011; Effects of a novel ultrasound contrast agent with long persistence on right ventricular pressure: comparison with SonoVue. Ultrasonics. 51:210–4. DOI: 10.1016/j.ultras.2010.07.008. PMID: 20825961.


5. Yang D, Tan KB, Gao YH, Liu H, Yang WX. 2012; Effects of diagnostic ultrasound-targeted microbubble destruction on permeability of normal liver in rats. Ultrasonics. 52:1065–71. DOI: 10.1016/j.ultras.2012.09.002. PMID: 23021237.


6. Wang G, Zhuo Z, Xia H, Zhang Y, He Y, Tan W, et al. 2013; Investigation into the impact of diagnostic ultrasound with microbubbles on the capillary permeability of rat hepatomas. Ultrasound Med Biol. 39:628–37. DOI: 10.1016/j.ultrasmedbio.2012.11.004. PMID: 23415284.


7. Chen ZY, Wang YX, Zhao YZ, Yang F, Liu JB, Lin Y, et al. 2014; Apoptosis induction by ultrasound and microbubble mediated drug delivery and gene therapy. Curr Mol Med. 14:723–36. DOI: 10.2174/1566524014666140804165245. PMID: 25088226.


8. Maeda H, Wu J, Sawa T, Matsumura Y, Hori K. 2000; Tumor vascular permeability and the EPR effect in macromolecular therapeutics: a review. J Control Release. 65:271–84. DOI: 10.1016/S0168-3659(99)00248-5. PMID: 10699287.


9. Nguyen AT, Wrenn SP. 2014; Acoustically active liposome-nanobubble complexes for enhanced ultrasonic imaging and ultrasound-triggered drug delivery. Wiley Interdiscip Rev Nanomed Nanobiotechnol. 6:316–25. DOI: 10.1002/wnan.1255. PMID: 24459007.


10. Min HS, You DG, Son S, Jeon S, Park JH, Lee S, et al. 2015; Echogenic glycol chitosan nanoparticles for ultrasound-triggered cancer theranostics. Theranostics. 5:1402–18. DOI: 10.7150/thno.13099. PMID: 26681985. PMCID: PMC4672021.


11. El-Dakdouki MH, Xia J, Zhu DC, Kavunja H, Grieshaber J, O'Reilly S, et al. 2014; Assessing the in vivo efficacy of doxorubicin loaded hyaluronan nanoparticles. ACS Appl Mater Interfaces. 6:697–705. DOI: 10.1021/am404946v. PMID: 24308364. PMCID: PMC3912576.


12. Sastry CS, Lingeswara Rao JS. 1996; Determination of doxorubicin hydrochloride by visible spectrophotometry. Talanta. 43:1827–35. DOI: 10.1016/0039-9140(96)01932-7. PMID: 18966670.


13. Kremkau FW. 1979; Cancer therapy with ultrasound: a historical review. J Clin Ultrasound. 7:287–300. DOI: 10.1002/jcu.1870070410. PMID: 112118.


14. Chen H, Hwang JH. 2013; Ultrasound-targeted microbubble destruction for chemotherapeutic drug delivery to solid tumors. J Ther Ultrasound. 1:10. DOI: 10.1186/2050-5736-1-10. PMID: 25512858. PMCID: PMC4265893.


15. Lin CY, Huang YL, Li JR, Chang FH, Lin WL. 2010; Effects of focused ultrasound and microbubbles on the vascular permeability of nanoparticles delivered into mouse tumors. Ultrasound Med Biol. 36:1460–9. DOI: 10.1016/j.ultrasmedbio.2010.06.003. PMID: 20800173.


16. Mizushige K, Kondo I, Ohmori K, Hirao K, Matsuo H. 1999; Enhancement of ultrasound-accelerated thrombolysis by echo contrast agents: dependence on microbubble structure. Ultrasound Med Biol. 25:1431–7. DOI: 10.1016/S0301-5629(99)00095-2. PMID: 10626631.


17. Vedantham K, Swet JH, McKillop IH, El-Ghannam A. 2012; Evaluation of a bioresorbable drug delivery system for the treatment of hepatocellular carcinoma. J Biomed Mater Res A. 100:432–40. DOI: 10.1002/jbm.a.33228. PMID: 22105845.


18. Lencioni R. 2012; Chemoembolization for hepatocellular carci-noma. Semin Oncol. 39:503–9. DOI: 10.1053/j.seminoncol.2012.05.004. PMID: 22846867.


19. May BJ, Murthy R, Madoff DC. 2012; What's new in transarterial therapies for hepatocellular carcinoma? Gastrointest Cancer Res. 5(3 Suppl 1):S14–9.
20. Cochran MC, Eisenbrey JR, Soulen MC, Schultz SM, Ouma RO, White SB, et al. 2011; Disposition of ultrasound sensitive polymeric drug carrier in a rat hepatocellular carcinoma model. Acad Radiol. 18:1341–8. DOI: 10.1016/j.acra.2011.06.013. PMID: 21971256. PMCID: PMC3188389.


21. Bekeredjian R, Kroll RD, Fein E, Tinkov S, Coester C, Winter G, et al. 2007; Ultrasound targeted microbubble destruction increases capillary permeability in hepatomas. Ultrasound Med Biol. 33:1592–8. DOI: 10.1016/j.ultrasmedbio.2007.05.003. PMID: 17618040.


22. Yin T, Wang P, Zheng R, Zheng B, Cheng D, Zhang X, et al. 2012; Nanobubbles for enhanced ultrasound imaging of tumors. Int J Nanomedicine. 7:895–904. DOI: 10.2147/IJN.S28830. PMID: 22393289. PMCID: PMC3289446.
23. Kabalnov A, Klein D, Pelura T, Schutt E, Weers J. 1998; Dissolution of multicomponent microbubbles in the bloodstream: 1. Theory. Ultrasound Med Biol. 24:739–49. DOI: 10.1016/S0301-5629(98)00034-9. PMID: 9695277.


24. Klibanov AL. 2006; Microbubble contrast agents: targeted ultrasound imaging and ultrasound-assisted drug-delivery applications. Invest Radiol. 41:354–62. DOI: 10.1097/01.rli.0000199292.88189.0f. PMID: 16481920.
25. Kim JH, Kim YS, Kim S, Park JH, Kim K, Choi K, et al. 2006; Hydrophobically modified glycol chitosan nanoparticles as carriers for paclitaxel. J Control Release. 111:228–34. DOI: 10.1016/j.jconrel.2005.12.013. PMID: 16458988.


26. Rahman A, Ganjei A, Neefe JR. 1986; Comparative immunotoxicity of free doxorubicin and doxorubicin encapsulated in cardiolipin liposomes. Cancer Chemother Pharmacol. 16:28–34. DOI: 10.1007/BF00255282. PMID: 3484382.


27. De Cock I, Zagato E, Braeckmans K, Luan Y, de Jong N, De Smedt SC, et al. 2015; Ultrasound and microbubble mediated drug delivery: acoustic pressure as determinant for uptake via mem-brane pores or endocytosis. J Control Release. 197:20–8. DOI: 10.1016/j.jconrel.2014.10.031. PMID: 25449801.


28. Li H, Wang J, Huang G, Wang P, Zheng R, Zhang C, et al. 2013; Multifunctionalized microbubbles for cancer diagnosis and therapy. Anticancer Agents Med Chem. 13:403–13. DOI: 10.2174/1871520611313030004. PMID: 23092268.


29. Tartis MS, McCallan J, Lum AF, LaBell R, Stieger SM, Matsunaga TO, et al. 2006; Therapeutic effects of paclitaxel-containing ultrasound contrast agents. Ultrasound Med Biol. 32:1771–80. DOI: 10.1016/j.ultrasmedbio.2006.03.017. PMID: 17112963.


30. Cai WB, Yang HL, Zhang J, Yin JK, Yang YL, Yuan LJ, et al. 2015; The optimized fabrication of nanobubbles as ultrasound contrast agents for tumor imaging. Sci Rep. 5:13725. DOI: 10.1038/srep13725. PMID: 26333917. PMCID: PMC4558543.


Fig. 2
Transmission electron microscope image (A) and size distribution (B) of echogenic nanobubbles.
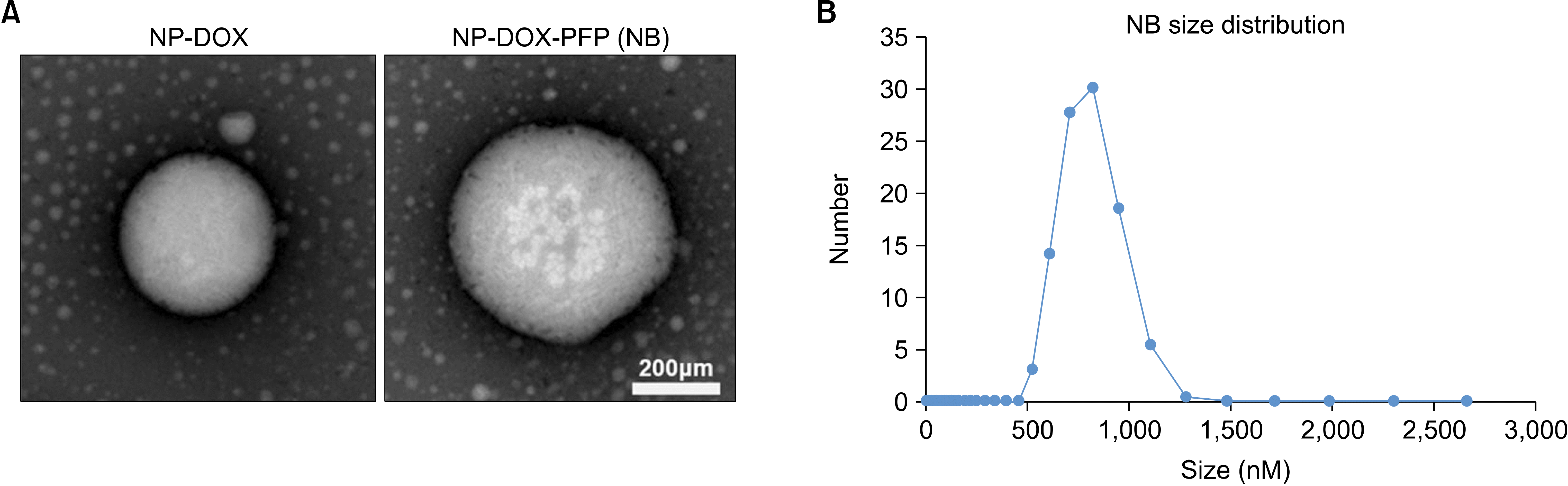
Fig. 3
View of experiment. After general anesthesia, the rat was placed in supine position. The abdominal wall was prepared for the experiment. a: ultrasound probe, b: inhalation mask.
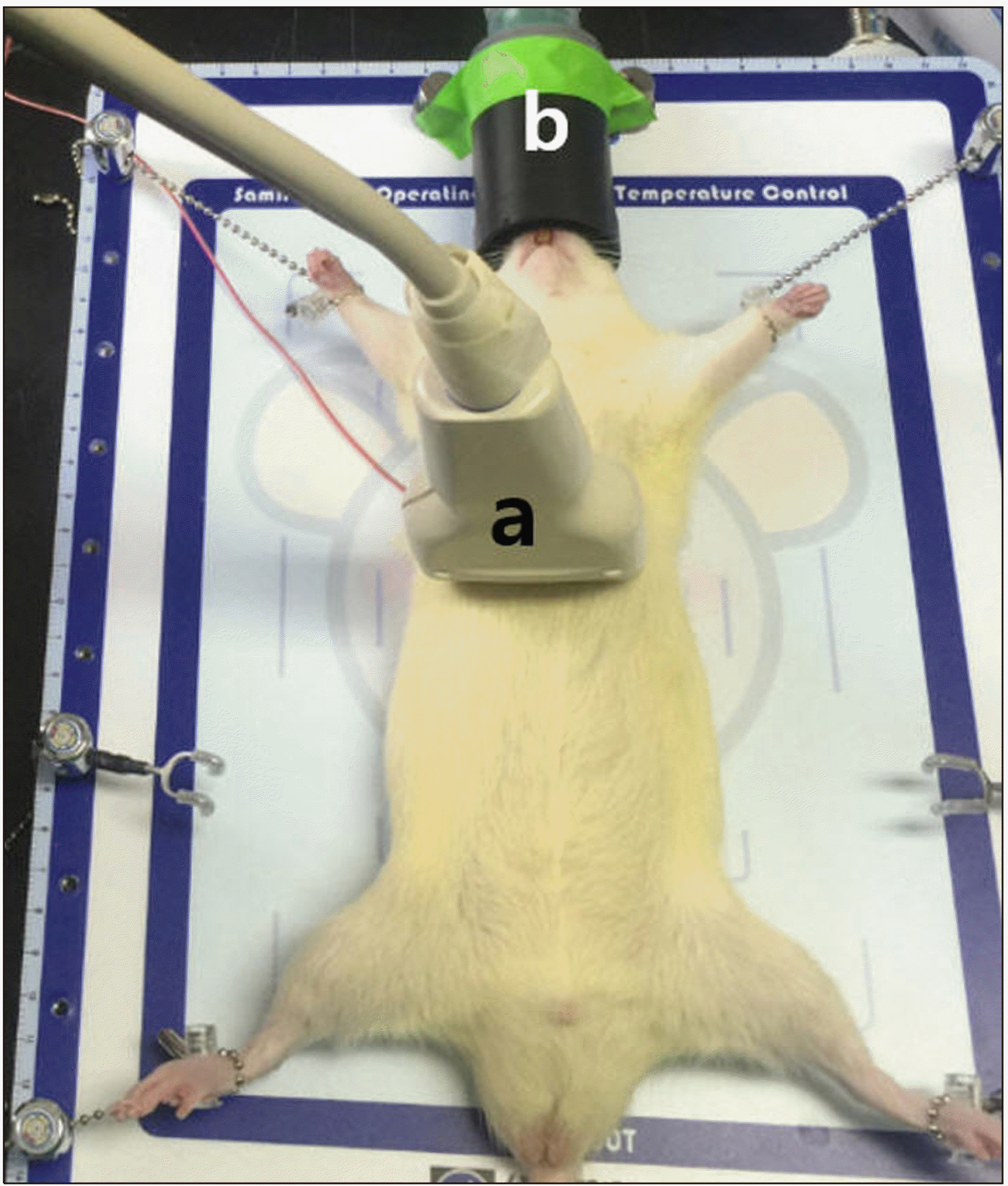
Fig. 4
Sonographic images of the rat liver. (A) no ultrasound group, (B) 5 MHz group, (C) 10 MHz group, (D) 13 MHz group, IVC: inferior vena cava, A: aorta, HV: hepatic vein.

Fig. 7
Change in distribution of fluorescence efficiency in organs after 5 MHz ultrasound irradiation.
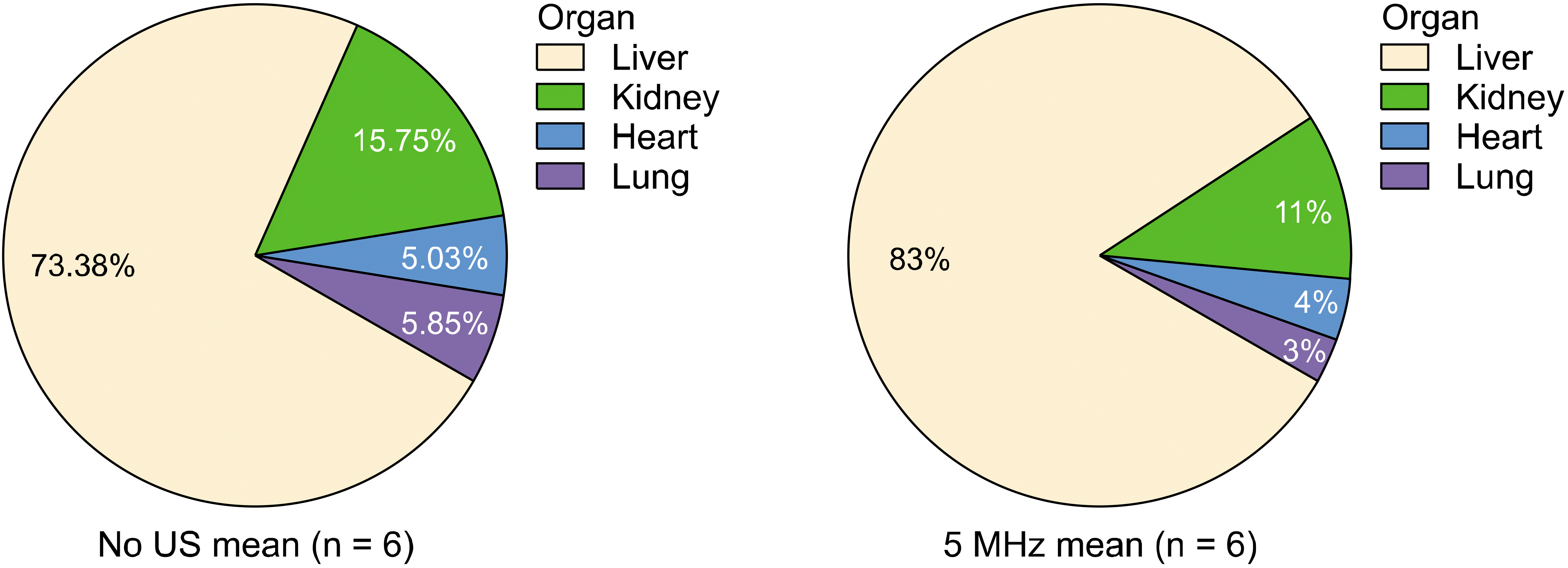
Table 1
Fluorescence Efficiency according to Frequency (scale = 108 [p/s]/[μω/cm2])
Table 2
Fluorescence Efficiency according to Duration of Ultrasound Irradiation (scale = 108 [p/s]/[μω/cm2])
Table 3
Laboratory Findings according to Duration of Ultrasound Irradiation
Table 4
Laboratory Findings according to Frequency of Ultrasound Irradiation