Abstract
Background and Objectives
Glioblastoma (GBM) is an aggressive primary brain tumor characterized by its heterogeneity and high recurrence and lethality rates. Glioblastoma stem cells (GSCs) play a crucial role in therapy resistance and tumor recurrence. Therefore, targeting GSCs is a key objective in developing effective treatments for GBM. The role of Parathyroid hormone-related peptide (PTHrP) in GBM and its impact on GSCs remains unclear. This study aimed to investigate the effect of PTHrP on GSCs and its potential as a therapeutic target for GBM.
Methods and Results
Using the Cancer Genome Atlas (TCGA) database, we found higher expression of PTHrP in GBM, which correlated inversely with survival. GSCs were established from three human GBM samples obtained after surgical resection. Exposure to recombinant human PTHrP protein (rPTHrP) at different concentrations significantly enhanced GSCs viability. Knockdown of PTHrP using target-specific siRNA (siPTHrP) inhibited tumorsphere formation and reduced the number of BrdU-positive cells. In an orthotopic xenograft mouse model, suppression of PTHrP expression led to significant inhibition of tumor growth. The addition of rPTHrP in the growth medium counteracted the antiproliferative effect of siPTHrP. Further investigation revealed that PTHrP increased cAMP concentration and activated the PKA signaling pathway. Treatment with forskolin, an adenylyl cyclase activator, nullified the antiproliferative effect of siPTHrP.
Glioblastoma (GBM), the highest grade IV astrocytoma, is the most common primitive malignant brain tumor and exhibits rapid progression, high metastasis, and fast relapse (1). Although the treatments for GBM (such as surgical resection, radiotherapy, and chemotherapy) have already achieved significant improvement, 5-year survival rates for patients with GBM remain poor (nearly 3%∼5%) (2). Previous studies have identified the presence of stem cell-like cells in GBM, termed glioblastoma stem cells (GSCs). These cells are strongly associated with intratumoral heterogeneity, therapy resistance, and GBM recurrence (3, 4). Therefore, novel effective therapies should be developed for GSCs.
As a member of the parathyroid family of hormones, parathyroid hormone-related peptide (PTHrP) is a highly conserved gene and expressed in a wide range of tissues, including bone, stomach, pancreas, lungs, etc (5). Previous studies demonstrated that PTHrP participated in the proliferation, differentiation, migration, and apoptosis of various types of stem cells (6). In human mesenchymal stem cells (MSCs), the addition of PTHrP could increase bone formation and decrease bone resorption (7). Bone morphogenetic protein 2 (BMP2) facilitated osteogenic differen-tiation in dental follicle stem cells via endogenous expression of PTHrP (8). Moreover, repression of PTHrP expression in neural stem cells (NSCs) inhibited prolifera-tion and differentiation and promoted apoptosis (9). PTHrP is essential not only for stem cells but also for different types of tumor cell progression. For example, PTHrP correlates with increased survival in patients with non-small-cell lung cancer and could be considered as a prognostic indicator (10). In breast cancer, PTHrP plays key roles in progression and metastasis. It is expressed in over 90% of bone metastatic breast cancers and 60% of primary breast tumors (11). However, we need to realize that there are conflicting reports in the published literature regarding the PTHrP function in breast cancer. One study showed that repression of PTHrP resulted in poorer outcomes (12), and the other concluded the opposite, that PTHrP promotes both tumor initiation and progression (13). Nonetheless, PTHrP may implicate a novel target to regulate tumor progression, especially cancer stem cells (CSCs). Following this hypothesis, we suspect that PTHrP is involved in regulating GSCs proliferation.
In this study, we first investigated the expression level of PTHrP association with patient survival and GBM ma-lignancy. Patient-derived GSCs was dissected from three surgical samples and these cells were used for subsequent experiments. Small interfering RNA (siRNA) was synthesized to inhibit PTHrP expression. Then, we investigated the effects of PTHrP on GSCs proliferation. Moreover, we also explored the underlying mechanisms of PTHrP regulation of GSCs proliferation, and the results suggested that the cAMP/PKA signaling pathway might be involved. Our present study may provide novel insight into the regulation of GSCs proliferation and offer a vigorous thera-peutic strategy for GBM treatment.
RNA sequencing (RNA-seq) data were downloaded from The Cancer Genome Atlas (TCGA) Data Matrix portal (Level3, https://tcga-data.nci.nih.gov/tcga/dataAccessMatrix.htm). Data were analyzed using the UCSC Xena browser (https://xenabrowser.net/), an easy-to-use cancer genomics visualization tool that analyzed 513 low-grade glioma (LGG) and 153 GBM samples. Log2 (normal count +1) was calculated using the scaled estimated expression values of 20,531 genes. Kaplan–Meier survival curves were analyzed using the UCSC Xena browser.
GBM tissues were collected from three post-surgical samples (GSC-01, GSC-02, and GSC-03) at the Depart-ment of Neurosurgery, the Second Affiliated Hospital of the Xi’an Jiaotong University. None of the patients underwent therapy prior to the surgical removal of the tumor. The principles of the Declaration of Helsinki were strictly followed, and clinical samples were obtained with infor-med consent. The detailed information is presented in Fig. 1C. Patient-derived GSCs were isolated as previously described and with minor modifications (14, 15). Briefly, the fresh GBM tissues were rinsed three times with pre-cooled Dulbecco’s Modified Eagle Medium/Nutrient Mixture F-12 (DMEM/F-12, Gibco). Then, the tissues were minced with autoclaved scissors and incubated with the dissociation medium (1 ml Dispase II (20 mg/ml, Sigma), 1 ml Collagenase type I (10 mg/ml, Sigma), and 8 ml DMEM/F12 medium) at 37℃ for 12 min. Cells were dissociated with triturated using a pipette and subsequently filtered using a 40 μm cell strainer (BD Falcon). The suspension was centrifuged at 1,000 rpm for 3 min at 4℃. Single cells (200,000 cells/ml) were plated in non-adhesive T25 flasks with complete medium (2 ml B27 (minus vitamin A), 1 ml N2, 20 ng/ml EGF, 10 ng/ml bFGF, 1 ml Pen Strep and 96 ml DMEM/F12 medium). Cells were incubated at 37℃ in a humidity incubator (Thermo Fisher) with 5% CO2/95% air. After culturing for 5∼7 days, 80∼200 μm spheres were observed in the medium. For single-cell adhesive culture, the spheres were dissociated into single cells using ACCUTASETM (Stem-cell Technologies, Canada) and plated onto poly-D-lysine-coated cell climbing slices or plates.
Cultured patient-derived GSCs were fixed with 4% paraformaldehyde (PFA) for 30 min at room temperature followed by washing three times with 0.01 M PBS. Cells were permeabilized using 0.1% Triton X-100 for 15 min. Then, cells were rinsed and blocked with a blocking buffer (5% bovine serum albumin and 5% horse serum) for 2 h at room temperature. The cells were incubated with the primary antibodies overnight at 4℃. After rinsing with 0.01 M PBS, samples were incubated with corresponding anti-IgG secondary antibodies. The information of the first and secondary antibodies were shown in Supplementary Table S1. The nucleus were counterstained with DAPI-containing mounting medium (Vector, USA). The sphere images were taken with a Leica SP8 confocal microscope, and the single-cell images were acquired using a fluorescence microscope (BX51, Olympus, Japan).
The human PTHrP-specific small interfering RNA (si-PTHrP) and scrambled sequence (siNC) were synthesized by Genepharma (Shanghai, China). The sequences were as follows:
siPTHrP: 5’-GACGAUUCUUCCUUCACCAdTdT-3’;
siNC: 5’-GACGAGCGGCACGUGCACATT-3’.
siRNA transfection was performed as previously described and with minor modification (16). Briefly, cells were plated on PDL-coated plates. After culturing for two days, lipofectamine 2000 transfection reagent (Invitrogen) was used to transfect siRNA (100 nM). For determining the transfection efficiency, cells were transfected with the fluorescently labeled siNC, and the normal siNC was used for most of the subsequent experiments. On the next day, the fluorescence was monitored on an inverted microscope system (DMI3000B, Leica). The knockdown efficiency was further detected by Western blot. The lentivirus vector containing shRNA targeting human PTHrP (KD-PTHrP) or negative control vectors (shNC) was purchased from Genechem. Cells (2×104 cells/well) were seeded in a 24-well plate and infected with 2 μl shUTX (1×108 virus, MOI=1:10) or 1 μl shNC (1×108 virus, MOI=1:5), respectively. One day later, the infected cells were selected with puromycin (1.6 μg/ml) for 24 hours.
5-week-old BALB/c nude male mice (20∼25 g) were purchased from the Experimental Animal Center of Xi’an Jiaotong University Health Science Center (Certificate No. 22–9601018). Mice were randomly assigned to two groups (5 mice/group) and were housed in a standard environment with ad libitum access to food and water. Mice were anesthetized with inhalational anesthesia using 4% isoflurane for induction and 1.5% isoflurane for mainte-nance. Then, mice were placed in a stereotactic frame (RWD LifeScience, China) and a longitudinal incision in the cranium was made at the midline to expose the bre-gma. After drilling a small hole, stably transfected patient-derived GSCs (1×105/μl) were implanted into the right brain using the following coordinates: anterior-posterior, −1.1 mm posterior to the bregma; medial-lateral, 1.2 mm; dorsal-ventral, 3.0 mm below the skull surface. The Hamilton syringe was performed slowly over a 1-min period at a speed of 5 μl/min. After the injection, the syringe was left in place for 5 min and the hole was sealed with bone wax. Mice were observed daily for signs of ill health. Mice were imaged using the IVISⓇ Spectrum In Vivo Imaging System (XENOGEN, USA) at day 3, 7 and 14, respec-tively.
Cells were incubated with BrdU (10 μg/ml) for 2 hours before fixation treatment. The BrdU-labeled cells were further visualized using immunostaining and normalized with propidium iodide (PI, Sigma-Aldrich) staining cells. Be-fore permeabilizing solution treatment, the cell climbing slices were pretreated with 2 N HCl for 30 min at 37℃, followed by neutralization in 0.1 M borate buffer (pH 8.5) for 15 min at room temperature.
Cell viability was measured by using the Cell Counting Kit‐8 (CCK‐8; 7sea, China) assay. Single patient-derived GSCs (3,000 cells/well) were inoculated into 96‐well plates followed by culturing for 1 day. Then, cells were treated with a serial concentration of recombinant human PTHrP protein (rPTHrP; 1, 3, 5, 10, 50, and 100 nM/ml, Sigma-Aldrich, USA) was added in the medium. After culturing for 3 days, 20 μl of CCK‐8 solution was added to each well and incubated for 2 hours at 37℃. The absorbance was measured using a multimicroplate spectrophotometer at 490 nm (BioTek, USA). Triplicate parallel wells were performed in all the experiments, and the data were collected as the average of at least three independent experiments. The results are presented as the absorbance value.
Protein extraction of cultured patient-derived GSCs was collected and lysed using a RIPA lysis buffer (Pierce) supplemented with a Protease Inhibitor Cocktail (Roche, Ger-many). The samples were sonicated (Sonics, USA) and centrifugated at 12,000 rpm for 10 min at 4℃. Proteins were subjected to electrophoresis via 10%∼12% sodium dode-cyl sulfate-polyacrylamide (SDS-PAGE) gels and transfer-red to polyvinylidene fluoride (PVDF) membranes (BioRad, USA). The membranes were blocked with 5% non-fat milk for 2 h, followed by incubated in primary antibodies (Sup-plementary Table S1) overnight at 4℃. The membranes were incubated with HRP-conjugated anti-rabbit or anti-mouse IgG for 2 h at room temperature. After thoroughly rinsed, immunoreactive bands were detected using an enhanced chemiluminescent substrate (Pierce) and Fuji X-ray film (Japan). The bands were visualized with a gel imaging system (G: Box, Syngene, UK) and analyzed with ImageJ software (NIH, USA). The expression levels of target proteins were determined and normalized to the housekeeping β-Actin. To normalize the data, β-actin was used as a housekeeping protein.
Patient-derived GSCs was transfected with siPTHrP or siNC, and the equal volume medium was added to the control group. The next day, single cells were inoculated into 24‐well plates. Neurosphere diameter (3,000 cells/well) and number (10,000 cells/well) were recorded by using an inverted microscope (Olympus CKX41, Japan) on the 3rd, 5th, and 7th day (inoculation in the 24‐well plates was deemed as 0 day). The images were analyzed with ImageJ software.
The cells were inoculated into 96‐well plates. After culturing for one day, 50 nM/ml rPTHrP was supplemented in the siPTHrP group and half of the medium containing drug was fed on the second day. After culturing for 3 days, the intracellular cAMP contents in each sample were determined using a commercial radioimmunoassay kit (R&D Systems, USA) according to the manufacturer’s instruc-tions. The optical density of each well was determined using a microplate reader (Epoch, BioTek) set to 450 nm. Triplicate parallel wells were examined in all the experiments, and the data were collected as the average of at least five independent experiments. For the PKA kinase activity assay, sample preparation and enzyme activity assay were performed according to manufacturers’ instru-ctions (Abcam, UK). Absorbance was measured after the substrate was added for 40 min.
All of the data were reported as mean±SD from at least three independent experiments. All statistical analyses were performed using GraphPad Prism 5. Differences between groups were analyzed using one-way ANOVA, followed by Tukey’s post hoc test. The data are shown as mean±standard deviation, and p<0.05 was considered as the statistically significant difference.
First of all, we analyze the differential expression of PTHrP between normal brain tissues and glioblastoma multiform samples by using RNA-seq data from TCGA. Compared to the normal brain tissues, the PTHrP expression was increased in GBM and LGG (Fig. 1A). Fur-thermore, the high level of PTHrP expression was negatively correlated with overall survival (Fig. 1B). These results indicated that PTHrP was a deleterious factor and inhibited its expression might be developed for GBM treatment. GBM tumors are very heterogeneous tumors that contain both neoplastic and subpopulations of GSCs. A number of studies have indicated that GSCs were closely associated with the therapeutic resistance and clinical outcome (17). Therefore, we tried to investigate whether PTHrP participates in regulating GSCs proliferation. The patient-derived GSCs was dissected from three surgical samples (Fig. 1C and 1D). After culturing for 5∼7 days, 80∼200 μm spheres were observed in the medium (Fig. 1E). Double immunofluorescent staining revealed that these spheres expressed both CD133 and nestin (Fig. 1F). Moreover, the single-GSCs staining showed 95.3%±6.2% CD133-positive cells, of which 97.1%±5.8% expressed SOX2 (Fig. 1G). These results strongly indicated that these primary cultured cells were GSCs.
The effect of PTHrP on patient-derived GSCs on cell viability was examined by CCK-8 assay. GSCs which obtained from three patients were treated with different concentrations of rPTHrP (0, 1, 3, 5, 10, 50, and 100 nM/ml) for 3 days. The results showed that PTHrP treatment significantly increased cell viability in a dose-dependent manner (Fig. 2A∼C). Because there was little difference between 50 and 100 nM/ml, 50 nM/ml treatment for 3 days was applied in the following experiments. To further investigate the effect of PTHrP in GSCs, the sphere formation assay was performed. The expression of PTHrP was inhibited by small interfering RNA. The results showed that the synthetic siRNA could be transfected into GSCs with high efficiency (Fig. 2D), and significantly reduced PTHrP expression (Fig. 2E and 2F). Knockdown PTHrP significantly decreased the number of tumorspheres, with increased disparities over time (Fig. 2G∼I). Similar to the counting result, the mean diameter of the tumorsphere was also decreased after inhibiting PTHrP expression (Fig. 2J∼L). Subsequently, we examined the effect of PTHrP on tumor formation. Patient-derived GSCs were infected with shRNA targeting human PTHrP (KD-PTHrP) or negative control (shNC) lentivirus. Similar to the siRNA result, KD-PTHrP significantly inhibited PTHrP expression (Fig. 2M and 2N). These cells were used to established an orthotopic xenograft mouse model, and tumor burden was monitored by in vivo imaging of luminescence. Live animal imaging showed that the suppression of PTHrP expression significantly inhibited tumor growth (Fig. 2O and 2P).
In order to further define the proliferation role of PTHrP, BrdU incorporation assay was carried out. The prolifera-tion rate by BrdU incorporation per PI-positive cells revealed a significant decrease of BrdU incorporation in PTHrP knockdown GSCs. Interestingly, the rPTHrP supplement significantly abolished the inhibitory effect of PTHrP on GSCs proliferation (Fig. 3).
In searching for a signal pathway that mediates the biological effects of PTHrP on GSCs, we focused on cAMP, which is the canonical pathway associated with PTHrP receptor activation (18). Knockdown PTHrP significantly decreased cAMP concentration, which was restored in the rPTHrP supplement group (Fig. 4A∼C). In an aim to further specify the effect of PTHrP on cAMP, we measured the activation of PKA, which is one of the main effectors of cAMP. As we expect, inhibition of PTHrP expression suppressed PKA activation. However, rPTHrP-exposed GSCs had a higher level of PKA (Fig. 4D∼F). These phenomena suggested that PTHrP could elevate intracellular cAMP levels in patient-derived GSCs.
To further explain whether the role of PTHrP in GSCs may be regulated by the cAMP/PKA signaling pathway, 10 μM forskolin (an adenylyl cyclase activator) was used to treat patient-derived GSCs which has been transfected with siPTHrP. BrdU staining showed that the number of BrdU-positive cells was significantly decreased after transfecting with PTHrP-target siRNA, while forskolin treatment almost restored the proliferative ability of the siPTHrP cells (Fig. 5A and 5B). These results suggested that PTHrP may influence the proliferation of patient-derived GSCs by regulating the cAMP/PKA signaling pathway.
As NSCs participates in tissue brain development, GSCs is closely correlated with GBM initiation, progression, resistance, and recurrence (3). Therefore, elucidating the mechanism of regulation of GSCs should greatly help in developing GBM treatment strategies. We showed that PTHrP promoted the proliferation of patient-derived GSCs, as indicated by the increased number and mean diameter of tumorsphere and the number of BrdU-positive cells. Fur-thermore, the proliferation effect of PTHrP seems to be closely associated with increasing the intracellular concentration of cAMP and subsequently promoting PKA acti-vation. Our study provides evidence that PTHrP participates in the regulation of patient-derived GSCs proliferation and highlights the PTHrP as a potential therapeutic target of GBM. However, the proliferation mechanism of PTHrP regulating the activation of the cAMP/PKA signaling pathway in the GSCs remains open and merits further explo-ration. Compared to the CSCs which sorting from cancer cell lines, the patient-derived CSCs more closely mirrors actual practice (19). In the present study, we investigated the proliferation effect of PTHrP in the patient-derived GSCs, and this enhances the trustworthiness of our findings.
PTHrP contributes to the formation of osteoclasts and bone resorption via endocrine, autocrine, paracrine, and intracrine hormone mechanisms (20). Previous studies have suggested that hypercalcemia which is mediated by PTHrP was strongly associated with several malignancies, including 85% of solid organ malignancies and 14.8% of hematologic malignancies (21). Furthermore, PTHrP-me-diated hypercalcemia also was closely related to a poor prognosis with a median survival of less than 2 months (22). In this study, we also noted that PTHrP expression was higher in GBM and negatively correlated with overall survival. Therefore, it is reasonable to hypothesize that PTHrP may be served as a clinical diagnosis and progno-stic marker in GBM. However, we need to be aware of the controversial effect of PTHrP on tumor progression. Some of the studies have provided evidence to show that PTHrP plays a pro-oncogenic role (23), the others have shown the role of PTHrP as a tumor suppressor (24). Thus, the exact role of PTHrP in regulating GBM progression requires further investigation.
As the first discovered second messenger, cAMP is involved in intracellular signal transduction, and response affects a wide variety of physiological and pathological processes (25). There are three main downstream effector molecules of cAMP, including PKA, the exchange proteins directly activated by cAMP (EPACs, also known as cAMP-GEFs), and cyclic-nucleotide-gated ion channels. Among them, PKA plays an essential role in the regulation of tumor progression and is a potential therapeutic target for cancer therapy (26). In this study, we demonstrated that knockdown PTHrP by target-specific siRNA decreased the level of cAMP and suppressed PKA activation. More importantly, the effect of siPTHrP was abolished by exposing the adenylyl cyclase activator. Therefore, we propose that PTHrP contributes to the proliferation of patient-derived GSCs via the cAMP/PKA signaling pathway. A high concentration of PTHrP in the GSCs niche promotes adenylyl cyclase activity, improving the intracellular level of cAMP. Accompanied by increasing cAMP, the PKA is activated, which eventually leads to promote proliferation (Fig. 5C). As we know, the physiological and pathological process is regulated by a complex signaling network, rather than one signaling pathway alone. Cross-talks among signaling pathways comprise the interwoven biochemical network that controls cellular behaviors and amplifies signaling cascades, eventually impacting in a series of biological processes (27). The mechanism of PTHrP on patient-derived GSCs cannot be simply explained by activation of the cAMP/PKA signaling pathway. Whether other signal pathways which take part in the PTHrP-mediated cell proliferation remains to be further explained.
Supplementary data including one table can be found with this article online at https://doi.org/10.15283/ijsc22097.
References
1. Ahir BK, Engelhard HH, Lakka SS. 2020; Tumor development and angiogenesis in adult brain tumor: glioblastoma. Mol Neurobiol. 57:2461–2478. DOI: 10.1007/s12035-020-01892-8. PMCID: PMC7170819.


2. Zhang Z, Zheng X, Luan Y, Liu Y, Li X, Liu C, Lu H, Chen X, Liu Y. 2018; Activity of metabotropic glutamate receptor 4 suppresses proliferation and promotes apoptosis with inhibition of gli-1 in human glioblastoma cells. Front Neu-rosci. 12:320. DOI: 10.3389/fnins.2018.00320. PMID: 29867331. PMCID: PMC5962807.


3. Lathia JD, Mack SC, Mulkearns-Hubert EE, Valentim CL, Rich JN. 2015; Cancer stem cells in glioblastoma. Genes Dev. 29:1203–1217. DOI: 10.1101/gad.261982.115. PMID: 26109046. PMCID: PMC4495393.


4. Prager BC, Bhargava S, Mahadev V, Hubert CG, Rich JN. 2020; Glioblastoma stem cells: driving resilience through chaos. Trends Cancer. 6:223–235. DOI: 10.1016/j.trecan.2020.01.009. PMID: 32101725. PMCID: PMC8779821.


5. Frieling JS, Lynch CC. 2019; Proteolytic regulation of parathyroid hormone-related protein: functional implications for skeletal malignancy. Int J Mol Sci. 20:2814. DOI: 10.3390/ijms20112814. PMID: 31181800. PMCID: PMC6600663.


6. Lai NK, Martinez D. 2019; Physiological roles of parathyroid hormone-related protein. Acta Biomed. 90:510–516.
7. Kim JW, Park N, Kang J, Kim Y, Jung H, Rim YA, Ju JH. 2021; Increased potential of bone formation with the intravenous injection of a parathyroid hormone-related protein minicircle DNA vector. Int J Mol Sci. 22:9069. DOI: 10.3390/ijms22169069. PMID: 34445802. PMCID: PMC8396456.


8. Pieles O, Reichert TE, Morsczeck C. 2022; Protein kinase A is activated during bone morphogenetic protein 2-induced osteogenic differentiation of dental follicle stem cells via endogenous parathyroid hormone-related protein. Arch Oral Biol. 138:105409. DOI: 10.1016/j.archoralbio.2022.105409. PMID: 35338829.


9. Liu Y, Wang Q, Wang Q, Cui M, Jin Y, Wang R, Mao Z, Miao D, Karaplis AC, Zhang YP, Shields LBE, Shields CB, Zhang Y. 2021; Role of PTHrP nuclear localization and carboxyl terminus sequences in postnatal spinal cord development. Dev Neurobiol. 81:47–62. DOI: 10.1002/dneu.22798. PMID: 33275829.


10. Montgrain PR, Deftos LJ, Arenberg D, Tipps A, Quintana R, Carskadon S, Hastings RH. 2011; Prognostic implications of parathyroid hormone-related protein in males and females with non--small-cell lung cancer. Clin Lung Cancer. 12:197–205. DOI: 10.1016/j.cllc.2011.03.018. PMID: 21663864.


11. McCauley LK, Martin TJ. 2012; Twenty-five years of PTHrP progress: from cancer hormone to multifunctional cytokine. J Bone Miner Res. 27:1231–1239. DOI: 10.1002/jbmr.1617. PMID: 22549910. PMCID: PMC4871126.


12. Fleming NI, Trivett MK, George J, Slavin JL, Murray WK, Moseley JM, Anderson RL, Thomas DM. 2009; Parathyroid hormone-related protein protects against mammary tumor emergence and is associated with monocyte infiltration in ductal carcinoma in situ. Cancer Res. 69:7473–7479. Erratum in: Cancer Res 2009;69:8832. DOI: 10.1158/0008-5472.CAN-09-0194. PMID: 19723659.


13. Li J, Karaplis AC, Huang DC, Siegel PM, Camirand A, Yang XF, Muller WJ, Kremer R. 2011; PTHrP drives breast tumor initiation, progression, and metastasis in mice and is a potential therapy target. J Clin Invest. 121:4655–4669. DOI: 10.1172/JCI46134. PMID: 22056386. PMCID: PMC3225988.


14. Azari H, Millette S, Ansari S, Rahman M, Deleyrolle LP, Reynolds BA. 2011; Isolation and expansion of human glioblastoma multiforme tumor cells using the neurosphere assay. J Vis Exp. (56):e3633. DOI: 10.3791/3633-v.


15. Liu Y, Luan Y, Ma K, Zhang Z, Liu Y, Chen X. 2022; ISL1 promotes human glioblastoma-derived stem cells' self-renewal by activation of sonic hedgehog/GLI1 function. Stem Cells Dev. 31:258–268. DOI: 10.1089/scd.2021.0344. PMID: 35403434.


16. Zhang Z, Zheng X, Liu Y, Luan Y, Wang L, Zhao L, Zhang J, Tian Y, Lu H, Chen X, Liu Y. 2021; Activation of metabotropic glutamate receptor 4 regulates proliferation and neural differentiation in neural stem/progenitor cells of the rat subventricular zone and increases phosphatase and tensin homolog protein expression. J Neurochem. 156:465–480. DOI: 10.1111/jnc.14984. PMID: 32052426.


17. Osuka S, Van Meir EG. 2017; Overcoming therapeutic resistance in glioblastoma: the way forward. J Clin Invest. 127:415–426. DOI: 10.1172/JCI89587. PMID: 28145904. PMCID: PMC5272196.


18. Liu S, Jean-Alphonse FG, White AD, Wootten D, Sexton PM, Gardella TJ, Vilardaga JP, Gellman SH. 2019; Use of backbone modification to enlarge the spatiotemporal diversity of parathyroid hormone receptor-1 signaling via biased agonism. J Am Chem Soc. 141:14486–14490. DOI: 10.1021/jacs.9b04179. PMCID: PMC6930011.


19. Nagle PW, Plukker JTM, Muijs CT, van Luijk P, Coppes RP. 2018; Patient-derived tumor organoids for prediction of cancer treatment response. Semin Cancer Biol. 53:258–264. DOI: 10.1016/j.semcancer.2018.06.005. PMID: 29966678.


20. Wysolmerski JJ. 2012; Parathyroid hormone-related protein: an update. J Clin Endocrinol Metab. 97:2947–2956. DOI: 10.1210/jc.2012-2142. PMID: 22745236. PMCID: PMC3431578.


21. Jin J, Chung JO, Chung MY, Cho DH, Chung DJ. 2017; Clinical characteristics, causes and survival in 115 cancer patients with parathyroid hormone related protein-mediated hyper-calcemia. J Bone Metab. 24:249–255. DOI: 10.11005/jbm.2017.24.4.249. PMID: 29259965. PMCID: PMC5734951.


22. Donovan PJ, Achong N, Griffin K, Galligan J, Pretorius CJ, McLeod DS. 2015; PTHrP-mediated hypercalcemia: causes and survival in 138 patients. J Clin Endocrinol Metab. 100:2024–2029. Erratum in: J Clin Endocrinol Metab 2019; 104:2614. DOI: 10.1210/jc.2014-4250. PMID: 25719931.


23. Nakajima T, Konda Y, Kanai M, Izumi Y, Kanda N, Nanakin A, Kitazawa S, Chiba T. 2002; Prohormone convertase furin has a role in gastric cancer cell proliferation with parathyroid hormone-related peptide in a reciprocal manner. Dig Dis Sci. 47:2729–2737. DOI: 10.1023/A:1021005221934. PMID: 12498293.
24. Luparello C, Romanotto R, Tipa A, Sirchia R, Olmo N, López de Silanes I, Turnay J, Lizarbe MA, Stewart AF. 2001; Midregion parathyroid hormone-related protein inhibits growth and invasion in vitro and tumorigenesis in vivo of human breast cancer cells. J Bone Miner Res. 16:2173–2181. DOI: 10.1359/jbmr.2001.16.12.2173. PMID: 11760830.
25. Sassone-Corsi P. 2012; The cyclic AMP pathway. Cold Spring Harb Perspect Biol. 4:a011148. DOI: 10.1101/cshperspect.a011148. PMID: 23209152. PMCID: PMC3504441.


26. Naviglio S, Caraglia M, Abbruzzese A, Chiosi E, Di Gesto D, Marra M, Romano M, Sorrentino A, Sorvillo L, Spina A, Illiano G. 2009; Protein kinase A as a biological target in cancer therapy. Expert Opin Ther Targets. 13:83–92. DOI: 10.1517/14728220802602349. PMID: 19063708.


27. Vert G, Chory J. 2011; Crosstalk in cellular signaling: background noise or the real thing? Dev Cell. 21:985–991. DOI: 10.1016/j.devcel.2011.11.006. PMID: 22172668. PMCID: PMC3281494.


Fig. 1
Culture and immunostaining characterization of patient-derived GSCs. (A) Comparison of PTHrP mRNA expression in samples between GBM (n=153) and LGG (n=513). ***p<0.001 versus normal tissue. (B) Kaplan–Meier survival analysis of GBM and LGG patients of lower and higher levels of PTHrP-mRNA expression. Human GSCs were isolated from three post-surgical patients (GSC-01, GSC-02, and GSC-03), and the samples’ detailed information (C) and H&E staining (D) were shown. Scale bar=50 μm. (E) After culturing for 5∼7 days, 80∼200 μm tumorspheres were observed. Scale bar=200 μm. (F) These tumorspheres were demonstrated using CD133 and nestin. (G) The single patient-derived GSCs were identified by CD133 and SOX2. The square frames are enlarged to identify the colocalization of CD133 and SOX2 staining. Scale bar=50 μm.
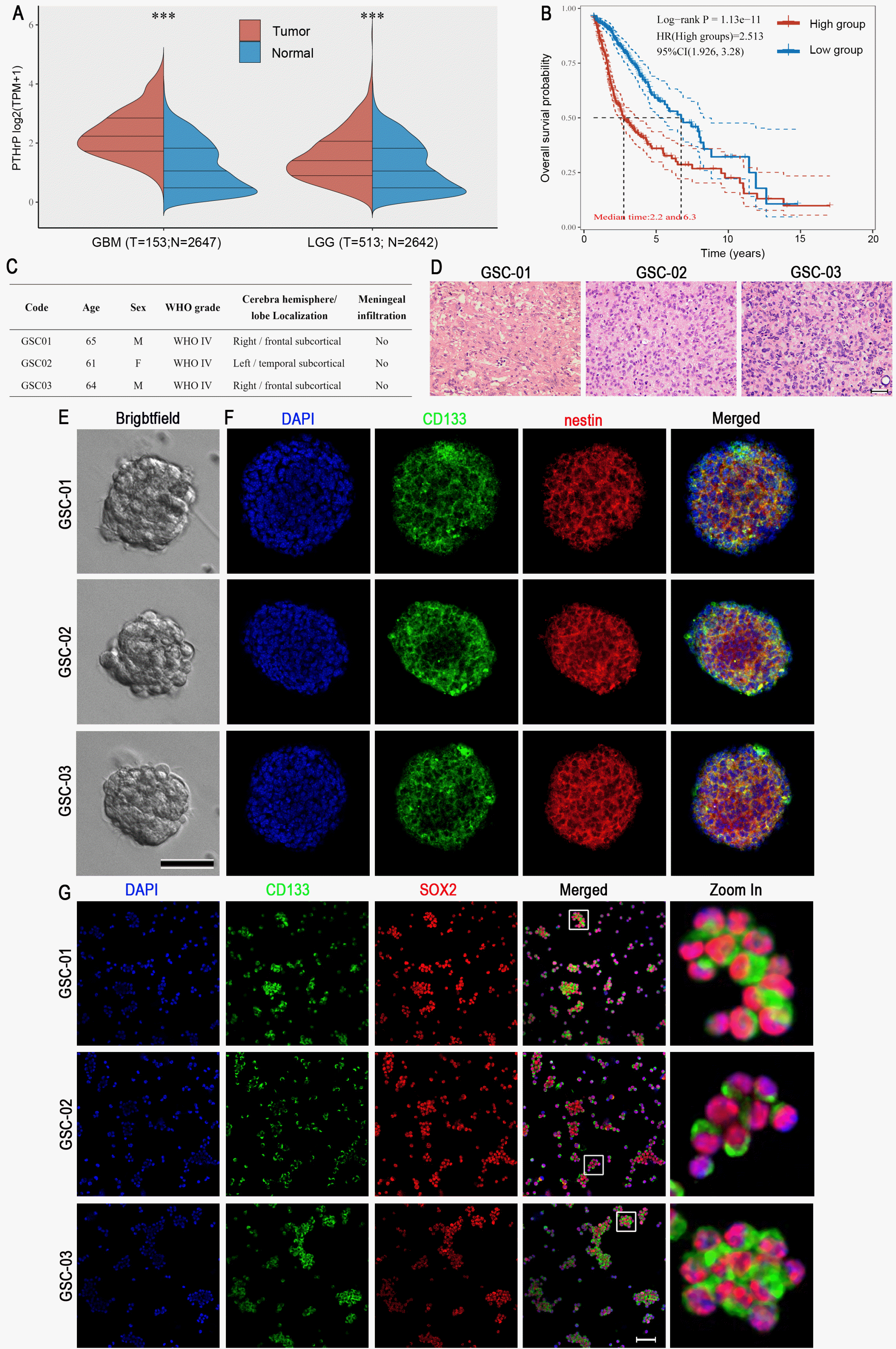
Fig. 2
PTHrP increases cell viability and tumorsphere formation of patient-derived GSCs. (A∼C) Three patient-derived GSCs (GSC-01, GSC-02, and GSC-03) were treated with a series of recombinant human PTHrP proteins (rPTHrP; 1, 3, 5, 10, 50, and 100 nM/ml) for 3 days, and cell viability was detected by CCK‐8 assay. *p<0.05, **p<0.01, ***p<0.001 versus control (Ctrl) group. (D) Patient-derived GSCs were transfected with FAM-labeled non-specific siRNAs (siNC) or PTHrP-targeted siRNA (siPTHrP). At the next day, more than 90% of transfected cells were observed. Scale bar=400 μm. (E, F) The expression of PTHrP was detected by western blot. Data from three independent experiments (n=3) were presented as the ratio of PTHrP to β‐Actin. ***p<0.001 versus the siNC group. After transfecting with siNC or siPTHrP, the tumorsphere number (G∼I) and the mean diameter (J∼L) were measured at 3 days, 5 days, and 7 days. Data were represented as the mean±SD of three independent experiments (n=3). (M, N) The PTHrP expression wsa examined by western blot. Data from three independent experiments (n=3) were presented as the ratio of PTHrP to β‐Actin. ***p<0.001 versus shNC group. The luciferase-expressing shNC GSCs or KD-PTHrP GSCs were used to establish the orthotopic glioma mouse model. (O, P) The in vivo imaging of shNC GSCs and KD-PTHrP GSCs in tumor-bearing mice at different time points after injection. Data are presented as the mean±standard deviation of five independent experiments (n=5). *p<0.05, **p<0.01 versus the shNC group.
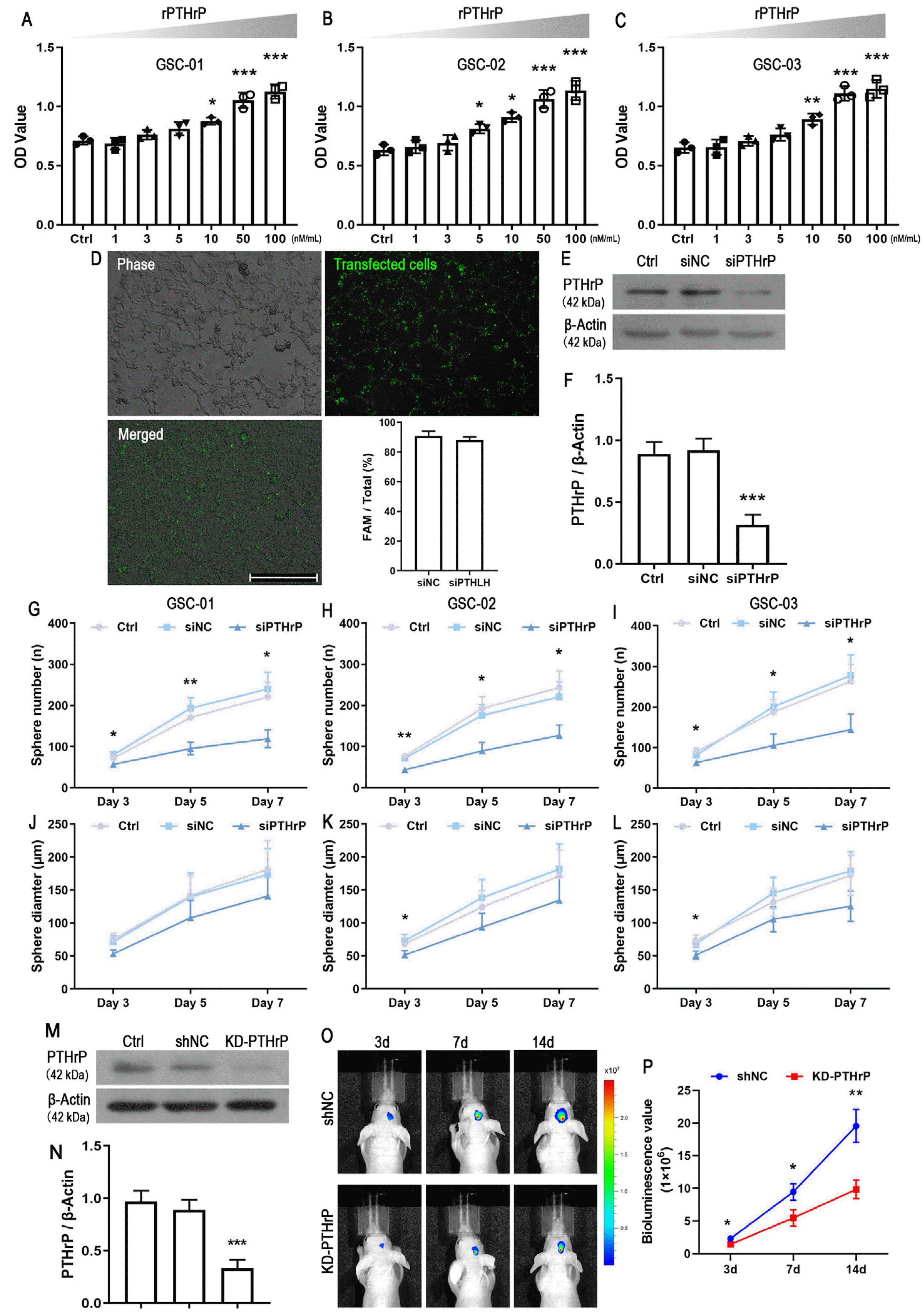
Fig. 3
Three patient-derived GSCs (GSC-01, GSC-02 and GSC-03) were transfected for 6 hours by siNC or siPTHrP. Then, knockdown PTHrP cells were exposed with 50 nM/ml rPTHrP or equal volume of the medium. (A) After culturing for 3 days, BrdU (10 mg/ml) incorporation assay was used for detecting proliferation cells. Scale bar=100 μm. (B) The value represents the mean±standard deviation of three independent experiments (n=3). *p<0.05 versus siNC group; ###p<0.001 versus siPTHrP group.
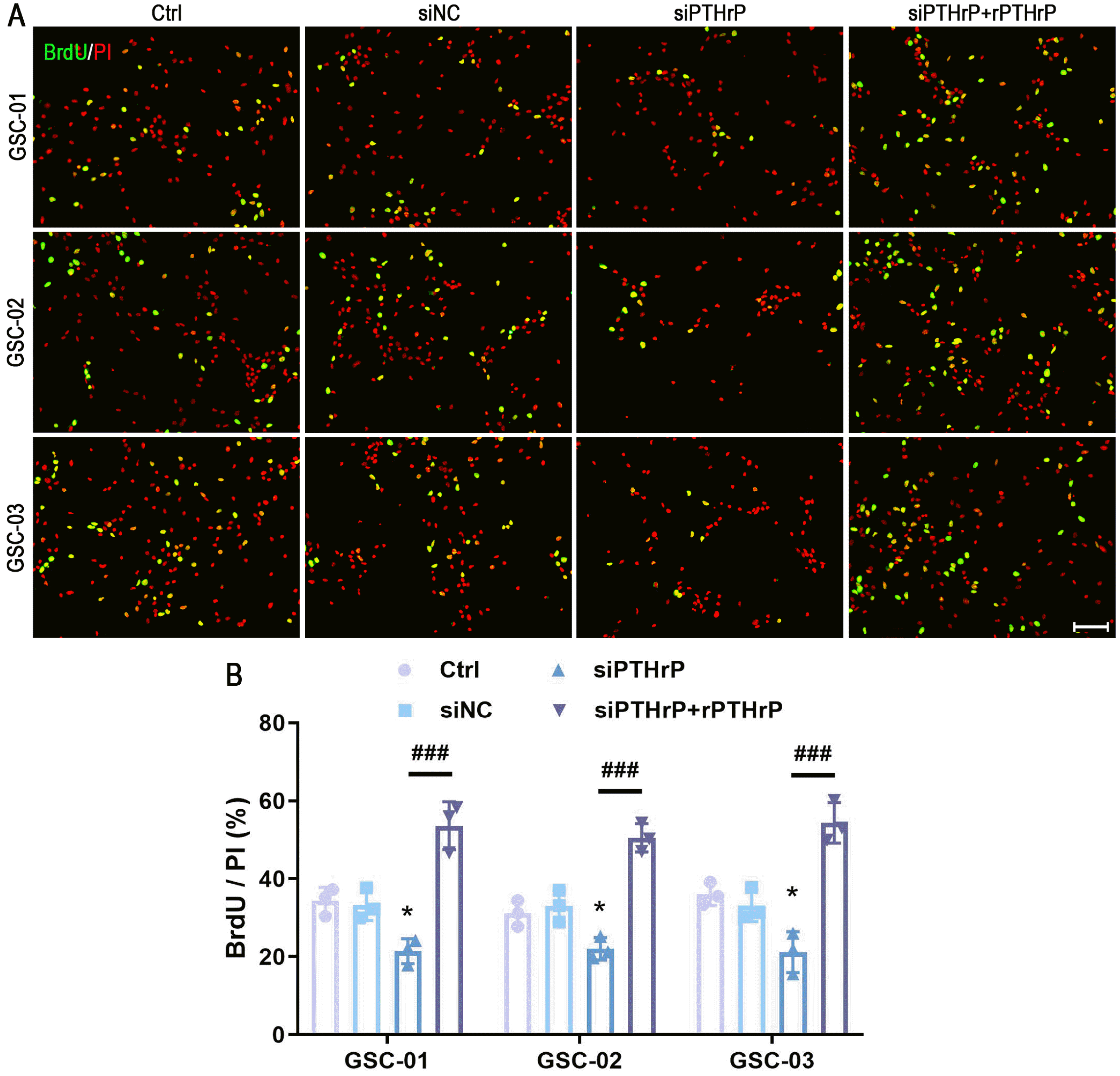
Fig. 4
PTHrP increases cAMP concentration and promotes PKA activation. After the transfection, cells were treated with 50 nM/ml rPTHrP for 3 days. The control group just contained the same volume of the medium. The concentration of cAMP (A∼C) and the PKA (D∼F) activity were measured by immunoassay and ELISA, respectively. The value represents the mean±standard deviation of five independent experiments (n=5). *p<0.05, **p<0.01 versus siNC group; ###p<0.001 versus siPTHrP group.
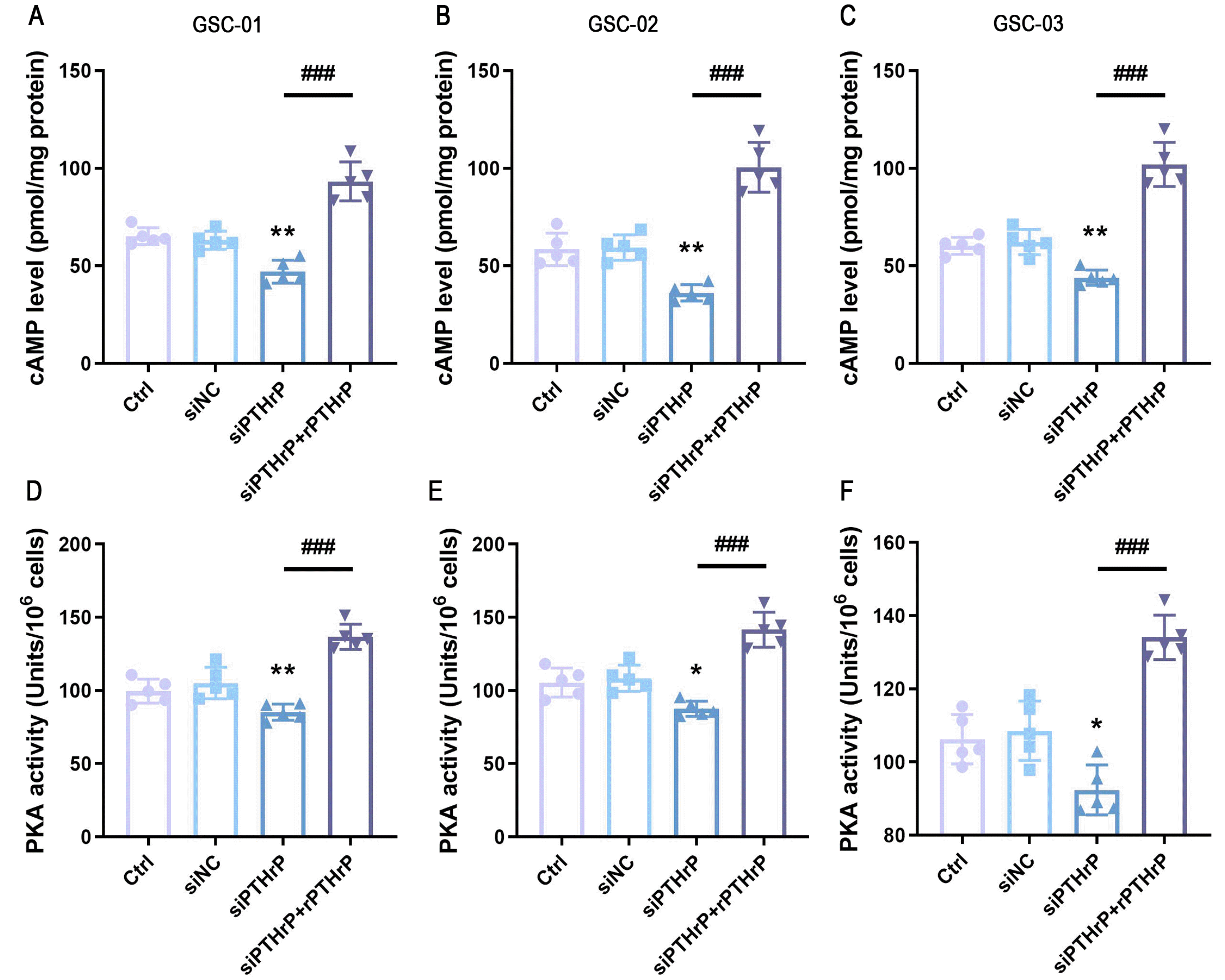
Fig. 5
cAMP/PKA signaling pathway is involved in regulating the proliferation effect of PTHrP in patient-derived GSCs. Three patient-derived GSCs (GSC-01, GSC-02, and GSC-03) were transfected with siNC or siPTHrP. The siPTHrP cells were randomly allocated into two groups. One group was treated with 10 μM forskolin, the other just add an equal volume of the medium. (A) After culturing for three days, cell proliferation was analyzed using BrdU incorporation. Scale bar=100 μm. (B) The value represents the mean±standard deviation of three independent experiments (n=3). *p<0.05 versus siNC group; ###p<0.001 versus siPTHrP group. (C) The illustration depicts the mechanism that PTHrP regulates the proliferation of patient-derived GSCs. PTHrP is secreted by GSCs to the extracellular environment. A high level of PTHrP significantly increases cAMP concentration via autocrine or paracrine routes. The PKA is activated due to a high level of cAMP, eventually promoting the proliferation of patient-derived GSCs. PTHR1: parathyroid hormone 1 receptor, PTHR2: parathyroid hormone 2 receptor, AC: adenylyl cyclase.
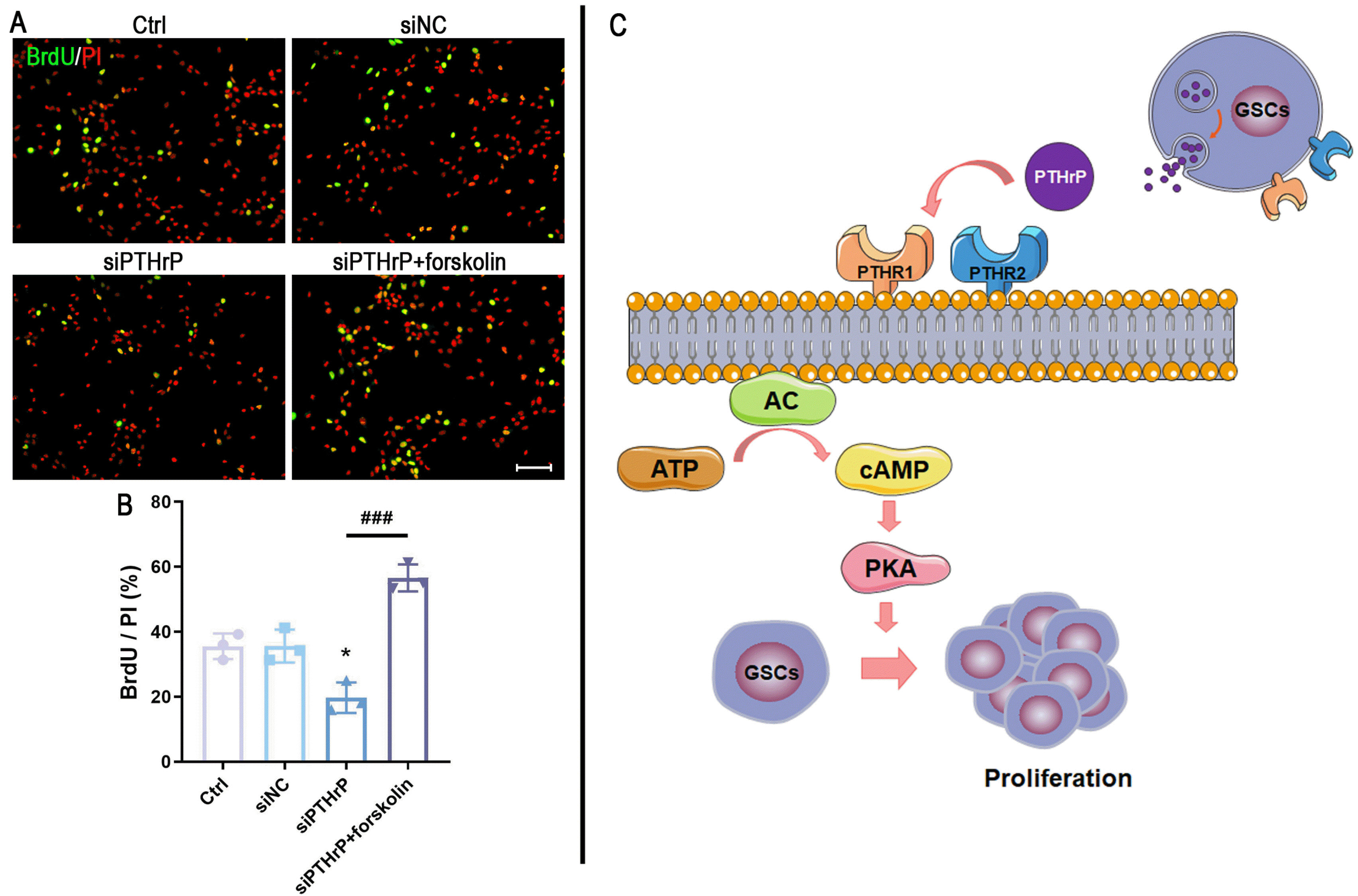