Abstract
Background and Objectives
Regenerative endodontic procedures (REPs) are a research hotspot in the endodontic field. One of the biggest problems of REPs is that it is difficult to realize regeneration of pulp-dentin complex and functional reconstruction. The reason is still not clear. We hypothesize that the migration may be different in different dental stem cells. Periodontal ligament stem cells (PDLSCs) may migrate faster than stem cells of apical papilla (SCAPs), differentiating into cementum-like tissue, bone-like tissue and periodontal ligament-like tissue and, finally affecting the outcomes of REPs. Hence, this study aimed to explore the mechanism that regulates the migration of PDLSCs.
Methods and Results
After isolating and culturing PDLSCs and SCAPs from human third molars, we compared the migration of PDLSCs and SCAPs. Then we investigated the role of SDF-1α-CXCR4/CXCR7 axis in PDLSC migration. We further investigated the impact of Porphyromonas gingivalis (P. gingivalis) lipopolysaccharide (LPS) on PDLSC migration and the potential mechanism. PDLSCs showed better migration under both noninflammatory and inflammatory conditions than SCAPs. SDF-1α can promote the migration of PDLSCs by elevating the expression of CXCR4 and CXCR7, increasing the interaction between them, promoting expression of β-arrestin1 and activating the ERK signaling pathway. P. gingivalis LPS can promote the migration of PDLSCs toward SDF-1α through increasing the expression of CXCR4 via the NF-κB signaling pathway, promoting the expression of β-arrestin1, and activating the ERK signaling pathway.
Pulp and periapical infection are diseases caused by microbial infection (1). Realizing pulp regeneration and functional reconstruction are the ultimate goals of the endodontic treatment, which is both a hot and difficult subject in endodontic field. Regenerative endodontic procedures (REPs) have been defined as biologically based procedures designed to replace damaged structures such as dentin, root structures, and cells of the pulp-dentin complex (2). This new treatment strategy has emerged as an alternative that aims to realize the healing of apical periodontitis and promoting pulpal physiologic functions. This treatment promotes continued root development, immune competency, and normal nociception, as shown in some published cases (3). Thus, the ultimate goal of these procedures is to regenerate the components and normal function of the pulp dentin complex.
However, REPs usually result in the formation of fibrous connective tissue, cementum-like tissue or bone-like tissue instead of pulp-dentin complexes (4-7). According to a systematic review of the histological assessment of REPs, only 2% (6/340) of studies reported the formation of dentin-like tissue after treatment, while most studies reported the formation of cementum-like tissue (53% [181/340]) and bone-like tissue (5% [17/340]) (8). These studies revealed that one of the problems of REPs is the difficulty of realizing regeneration of the complete dentin-pulp complex. The reason is still not clear.
Strategies to revitalize dental pulp include revascularization, cell-homing-based regeneration and cell-based regeneration (9). Revascularization and cell-homing-based regeneration rely on the regenerative potential of host stem/precursor cells (10), which are considered a relatively easy way to regenerate dental pulp tissue since there is no need to isolate stem/precursor cells from the donor and to expand them in vitro (11, 12). However, most studies that reported the formation of pulp regeneration applied the stem cell-based approach (8, 13, 14), while the cell-homing based approach and revascularization usually resulted in the formation of cementum-like tissue or bone-like tissue (4).
Several kinds of dental stem cells have been used in REPs, including dental pulp stem cells (DPSCs), stem cells from apical papilla (SCAPs), periodontal ligament stem cells (PDLSCs), dental follicle cells (DFCs) and stem cells isolated from human pulp of exfoliated deciduous teeth (SHEDs) (15). PDLSCs mainly differentiate into bone-like or cementum-like tissue (16) while SCAPs and DPSCs mainly differentiate into odontoblast-like cells (17). Although the stem cell niche may affect the differentiation potential of stem cells (18), some researchers found that the odontogenic differentiation potential of stem cells from other tissues is weak even in odontogenic differentiation environments (19). Since the first step of the cell-homing based approach and revascularization is stem cell migration, we presumed that the possible reason for the formation of cementum-like tissue or bone-like tissue after REPs is that the cells that mainly differentiate into bone-like or cementum-like tissue take the lead in entering the root canal and play a dominant role during REPs, i.e., PDLSCs may migrate faster than SCAPs or DPSCs. Thus, exploring the mechanism which regulates the migration of PDLSCs would provide some hints to interfere its migration and prevent the formation of cementum-like tissue or bone-like tissue during REPs.
However, microorganisms may also affect the outcomes of REPs. It was revealed that root canal preparation and chemical disinfection cannot completely kill the microorganisms or clear lipopolysaccharide (LPS) (20). Infectious substances such as LPS remaining in root canals may change the biological behavior of stem cells such as migration (21), proliferation (22) and differentiation (23), affecting the outcomes of REPs.
Furthermore, according to previous studies, stromal-de-rived factor 1α (SDF-1α, also known as CXCL12) and its receptor CXCR4 are important in stem/progenitor cell chemotaxis and migration to specific injury spots (24). However, the function of CXCR7, another receptor of SDF-1α with high affinity, is still not clear. Previous studies revealed that CXCR7 may function as a scavenger receptor that provides the proper SDF-1α concentration (25). Some studies have revealed that CXCR7 can regulate cell migration by interacting with SDF-1α (26). Other studies found that CXCR7 heterodimerizes with CXCR4 and regulates CXCL12-mediated cell migration (27, 28). The specific function of CXCR7 in PDLSC migration is still not clear.
In conclusion, propose the following: 1) The migration ability may be different in different dental stem cells. PDLSCs may first enter the root canal space. 2) The remaining infectious substances (LPS) in root canals may influence the migration of different kinds of stem cells by affecting the expression of CXCR4 and CXCR7 on the cell surface. Hence, in this study, we first compared the differences in migration between PDLSCs and SCAPs. Then, we mainly focused on PDLSCs and investigated the role of the SDF-1α-CXCR4/CXCR7 axis in PDLSC migration under noninflammatory and inflammatory conditions.
This study was approved by the university clinical research ethics board (WCHSIRB-2020-366). Patients with chief complaint of third molar extraction, aged from 18∼20 years old, without periodontal diseases, oral mucosal diseases or systemic disease were included in this study. Healthy and intact human third molars with open apex were collected from them. Teeth with cracks, caries, coronal fillings or root external defects were excluded. Teeth were soaked in prechilled antibiotic PBS solution (100 U/ml penicillin and 100 mg/ml streptomycin). PDLSCs and SCAPs were isolated from extracted healthy and intact human third molars with open apexes from various individuals. The periodontal tissue was scratched from the middle third of the root surface. The apical papilla tissue was cut from the open apex. These tissue were respectively cut into pieces and cultured in Dulbecco’s modified Eagle’s medium (DMEM, Gibco, Wien, Austria) containing 20% fetal bovine serum (Gibco), 50 mg/ml streptomycin, and 100 U/ml penicillin (Sigma‒Aldrich, MO, USA) at 37℃ in a humidified atmosphere of 5% CO2 and 95% air. Cells were passaged after reaching 80% confluence. The cells at the third to fifth passages were used in the following experiments.
For characterization of the multidirectional differentiation ability of cells, PDLSCs and SCAPs were incubated in osteogenic differentiation medium (α-MEM with 10% FBS, 10 nM dexamethasone, 10 mM β-glycero-phosphate, 50 μg/ml ascorbate phosphate, 10 nM 1,25-dihydroxyvitamin D3) or adipogenic differentiation medium (α-MEM with 10% FBS, 1 μM dexamethasone, 1 μg/ml insulin, 0.5 mM IBMX) for 4 weeks respectively. The osteogenic and adipogenic differentiation medium was replenished every 2∼3 days. At the end of the culture period, cells incubated in osteogenic differentiation medium were stained with 1% alizarin red S (Sigma‒Aldrich) for mineral deposits detection. Cells incubated in adipogenic differentiation medium were stained with oil red O (Sigma‒Aldrich) for oil droplet detection (29).
PDLSCs were pretreated with 100 ng/ml, 1 μg/ml, or 10 μg/ml P. gingivalis LPS for different times and then subjected to RNA extraction, quantitative real-time PCR (qPCR) assays, protein extraction and Western blotting (WB) assays.
For inhibition experiments, PDLSCs were pretreated with ERK inhibitor (10 μM SCH772984, 1 h; Selleck Chemicals, TX, USA), AKT inhibitor (5 μM MK-2206 2HCl, 1 h; Selleck Chemicals) or NF-κB inhibitor (CAS 354811-10-2, 4.75 μM, 6 h; Santa Cruz, TX, USA) and then subjected to WB. After confirming that ERK, AKT or NF-κB signaling pathway was blocked, the cells were subjected to a transwell assay to evaluate their migration.
For small interfering RNA transfection experiments, PDLSCs were transfected with 50 nmol/l control small interfering (siRNA) or specific CXCR4 siRNA, CXCR7 siRNA, ARRB1 siRNA, respectively (GenePharma, Shanghai, China) using Lipofectamine 2000 Transfection Reagent (Life Te-chnologies, CA, USA). The siRNA sequences used are listed in Table 1.
After 36 h of transfection, whole proteins were extracted from cells and subjected to WB. After the transfection efficiency was confirmed, cells were then subjected to transwell assay to evaluate the migration.
Cells of different groups (2×104 cells/200 μl) were seeded in the upper chamber, and 500 μl serum-free αMEM with 100 ng/ml SDF-1α (R&D Systems, MN, USA) was added to the lower chamber. Serum-free αMEM without SDF-1α were used as negative control. After incubation for 16 hours, the cells were washed with PBS for two times, fixed with 4% paraformaldehyde for 30 minutes, and incubated with 0.1% crystal violet for 20 minutes. Cotton wool was used to scrape off the nonmigrating cells in the upper chamber. Migrating cells were counted at 40-fold magnification in 5 random fields. The number of cells was counted using Image-Pro Plus 6.0 software (Media Cybernetics, MD, USA).
Total RNA was extracted from PDLSCs or SCAPs using TRIzol (Life Technologies, CA, USA). Complementary DNAs were synthesized from RNA using the PrimeScript RT Reagent Kit (TaKaRa, Tokyo, Japan) and subjected to quantitative polymerase chain reaction with the SYBR Premix Ex Taq II kit (TaKaRa) in the ABI 7300 Real-Time PCR System (Applied Biosystems, CA, USA) according to the manufacturer’s protocol.
The expression of targeted genes was normalized to the expression of glyceraldehyde-3-phosphate dehydrogenase. The primer sequences used are listed in Table 2.
Cells were washed with PBS for three times, and the cell lysates of PDLSCs or SCAPs were collected with cell lysis buffer (Pierce Biotech, IL, USA).
The whole proteins were extracted, loaded for electrophoresis using 10% sodium dodecyl sulfate‒polyacrylamide gel electrophoresis, and then transferred to polyvinylidene fluoride membranes (Roche Diagnostics GmbH, Mannheim, Germany). The membranes were blocked in 5% bovine serum albumin in Tris-buffered saline-0.1% Tween 20 (Beyo-time, Shanghai, China) for 1 hour and probed with primary antibodies against CXCR4 (1:100, Abcam), CXCR7 (1:1,000, R&D), phosphor-ERK1/2 (1:2,000, CST), ERK1/2 (1:1,000, CST), phosphor-AKT (1:1,000, CST), AKT (1:1,000, CST), β-arrestin 1 (1:1,000, CST), alpha tubulin (1:3,000, Abcam), phosphor-P65 (1:1,000, CST), P65 (1:1,000, CST) and GAPDH (1:5,000, Abcam), overnight at 4℃. The primary antibodies were detected with anti-rabbit or anti-mouse immunoglobulin G conjugated to horseradish peroxidase (1:5,000, SAB, Green-belt, Maryland, USA) for 1 hour at room temperature. Signals were captured using the ECL Plus Western Blotting Detection System (GE Healthcare, IL, USA). The images were then analyzed with ImageJ software (Wayne Rasband National Institutes of Health, USA).
Cells were lysed by IP buffer (Beyotime, China), incubated with anti CXCR4 (Abcam) or anti CXCR7 antibody (R&D) overnight at 4℃. Protein A/G magnetic beads (Bimake) were subsequently added and the mixture were incubated at 4℃ for 1 h. The beads were collected by magnetic separation and the non-binding supernatant was discarded. Bound protein was eluted by boiling at 100℃ for 5 min. Samples were subjected to Western blotting to evaluate the interaction of CXCR4 and CXCR7.
Human periapical lesions were collected during periradicular surgery. Normal gingival tissue and apical papilla tissues were collected from extracted healthy third molars as controls. Tissues were immediately fixed in 4% paraformaldehyde in PBS at 4℃ for 24 h, embedded in paraffin and cut into 5 μm-thick sections.
Hematoxylin-eosin staining was carried out to analyze the periapical lesion and normal tissue (gingiva tissue and apical papilla). Immunohistological staining was performed to evaluate the expression of CXCR4 and CXCR7 in different tissues. Immunohistochemistry was performed according to the instructions of SP-9001 kit (ZSGB-BIO, Beijing, China). Sections were subjected to epitope recovering in pancreatic enzymes by 0.1% (V/V) at 37℃ for 20 min. Then nonspecific immunoglobulin binding was blocked by 5% (V/V) bovine serum albumin for 30 min at 37℃. Then, the sections were incubated with anti-CXCR4 (Abcam) or anti-CXCR7 (R&D), overnight at 4℃. Finally, specimens were developed with a 3,3’-diaminobenzidine tetrahydrochloride chromogen kit (ZSGB-BIO) and counterstained with hematoxylin.
In addition, total RNA from different tissues was extracted and subjected to qPCR to evaluate the expression of CXCR4 and CXCR7.
PDLSCs and SCAPs were isolated from freshly extracted healthy human third molars. After culture for 1∼2 weeks, the colony formation of PDLSCs and SCAPs at passage 0 were observed. Both cell populations presented a homogeneous fibroblast-like morphology (Supplementary Fig. S1A and S1D). Mineral deposits were observed in PDLSCs and SCAPs after osteogenic induction for 4 weeks, indicating the potential ability of odonto/osteogenic differentiation in both cell lines (Supplementary Fig. S1B and S1E). Oil droplet-filled adipocyte-like cells were present in both groups after adipogenic induction for 4 weeks, showing the adipogenic differentiation (Supplementary Fig. S1C and S1F). Immunophenotypic analysis of PDLSCs and SCAPs showed that PDLSCs expressed the specific MSC antigens CD90, SCAPs expressed CD24, and both kinds of cells were negative for the hematopoietic marker CD45 (Supplementary Fig. S1G∼L).
Transwell assays were used to compare the migration of PDLSCs and SCAPs. According to the results, SDF-1α improved the migration of PDLSCs and SCAPs under normal conditions. There were more migrated PDLSCs than SCAPs regardless of whether they were stimulated with SDF-1α (Fig. 1A∼E). LPS improved the migration of PDLSCs and SCAPs in a concentration-dependent manner. There were more migrated PDLSCs than SCAPs, regardless of the LPS concentration (Fig. 1F∼N). Since PDLSCs presented stronger migration than SCAPs, we focused on PDLSCs in the following experiments.
qPCR assays revealed that SDF-1α increased the gene expression of CXCR4 and CXCR7 in PDLSCs (Fig. 2A and 2B). Then we verified the results by WB assays. SDF-1α increased the protein expression of CXCR4 and CXCR7 in a time-dependent manner (Fig. 2C∼E). These results confirmed that CXCR4 and CXCR7 may be related to the migration of PDLSCs.
To further investigate the potential role of SDF-1α-CXCR4/CXCR7, we conducted a coimmunoprecipitation (co-IP) assay. As shown in Fig. 2F, CXCR4 interacted with CXCR7, and this interaction was enhanced by SDF-1α stimulation. Then we transinfected PDLSCs with CXCR4 and CXCR7 siRNA (Fig. 2G∼J) and evaluated the impact on migration. The migrated PDLSCs induced by SDF-1α were significantly suppressed by CXCR4 siRNA or CXCR7 siRNA (Fig. 2K∼N).
To evaluate the signaling pathways induced by SDF-1α, we treated PDLSCs with SDF-1α (100 ng/ml) for 0∼120 minutes. WB results revealed that SDF-1α elevated the phosphorylation levels of ERK1/2 and AKT, as well as β-arrestin1. The p-ERK1/2 level was increased after 5∼120 minutes of exposure, peaking at 5 minutes, while the p-AKT level peaked at 30 minutes. The β-arrestin1 level was also increased and peaked at 30 min (Fig. 3A∼D).
To assess the role of the ERK and AKT signaling pathways in the migration of PDLSCs, we pretreated cells with an ERK inhibitor (SCH772984, 1 h; Selleck Chemicals, TX, USA) or AKT inhibitor (5 μM MK-2206 2HCl, 1 h; Selleck Chemicals, TX, USA). The suppression of β-ar-restin1 expression in PDLSCs was achieved by ARRB1 (gene encoding β-arrestin1 protein)-specific siRNA trans-fection. After inhibition of ERK signals (Fig. 3E) and AKT signals (Fig. 3I) or suppression of β-arrestin1 (Fig. 3M), the number of migrated PDLSCs was greatly reduced (Fig. 3F∼H, J∼L, N∼P).
Furthermore, after transfection with CXCR4 and CXCR7 siRNA, SDF-1α-induced p-ERK, p-AKT and β-arrestin1 upregulation was suppressed in PDLSCs (Fig. 3Q∼T). In the PDLSCs transfected with ARRB1 siRNA, p-ERK expression was also decreased (Fig. 3X∼Y). However, treatment with the ERK inhibitor increased the expression of β-arrestin1 in PDLSCs (Fig. 3U∼W).
To investigate the expression of CXCR4 and CXCR7 in clinical inflammatory conditions, we collected human apical lesion tissues during periradicular microsurgery and defined them as the “INFLAMMATION” group. Normal gingival tissues and apical papilla tissues were collected from extracted healthy third molars and defined as the “NORMAL” group. According to the results of hematoxylin and eosin staining, fibrous connective tissues were observed in normal gingiva tissues and apical papilla tissues. Inflammatory cell infiltration was observed in apical lesion tissues (Fig. 4Aa∼Ac). There was no positive expression of CXCR4 or CXCR7 in the NC groups by immunohistochemistry staining (Fig. 4Ad∼Af). More fibroblast-like cells with positive CXCR4 expression were present in apical lesion tissue (Fig. 4Ag∼Ai). CXCR7 was positively expressed in few cells in all tissues which mainly surrounded vessels. Almost no differences were shown between apical lesion tissues and normal tissues (Fig. 4Aj∼Al). Total RNA was extracted from specimens in the INFLAMMATION group (apical lesion tissues) and NORMAL group (gingival tissues and apical papilla tissues) and analyzed by qPCR assays. The expression of CXCR4 was higher in the INFLAMMATION group while the expression of CXCR7 was nearly the same in the two groups (Fig. 4B and 4C).
To investigate the mechanism of PDLSC migration under LPS stimulation, we pretreated PDLSCs with an NF-κB inhibitor (CAS 354811-10-2, 4.75 μM, 6 h), LPS (1 μg/ml, 48 h), and SDF-1α (100 ng/ml, 30 min). WB results showed that LPS improved p-P65 expression, which was reversed by NF-κB inhibitor treatment (Fig. 5A and 5B).
Transwell assays revealed that LPS improved the migration of PDLSCs, which was reversed by NF-κB inhibitor treatment (Fig. 5C∼F). Moreover, LPS-induced CXCR4 expression was suppressed by NF-κB inhibitor treatment. LPS and NF-κB inhibitor treatment did not affect the expression of CXCR7 (Fig. 5G∼I). LPS improved the expression of p-AKT, p-ERK and β-arrestin1, while NF-κB inhibitor treatment suppressed the above effect of LPS (Fig. 5J∼M).
Cell migration is the first step of revascularization and cell homing-based REPs. However, most studies comparing the differences among different dental stem cells investigated the differentiaon ability and stemness, instead of migration. According to this study, we found that PDLSCs showed stronger migration mediated by SDF-1α than SCAPs. Considering that the first step of the cell-homing-based approach and revascularization is stem cell migration, we presumed that PDLSCs, which mainly differentiate into the bone-like or cementum-like tissue may migrate first into root canal and play a dominant role during REPs.
Various studies have confirmed that SDF-1α-CXCR4/CXCR7 plays an important role in cell migration (27, 32-35). Therefore, we focused on this axis in the study. We found that SDF-1α can promote the migration of PDLSCs by elevating the expression of CXCR4 and CXCR7 and increasing the interaction of CXCR4 and CXCR7. Conventional studies suggested that CXCR4 can be coupled to Gαi, Gαq or Gα12/13, resulting in a complex signaling cascade involving the mitogen-activated protein kinase (MAPK), phospholipase C and phosphatidylinositol-3-kinase pathways and ending with cellular migration or activation of adhesion molecules (32, 33). Other studies supported that CXCR7 can promote cell migration by forming a heterodimer with CXCR4 or regulating signaling independent of CXCR4 after stimulation with SDF-1 (27, 34, 35). In our study, we found that CXCR7 can interact with CXCR4 and that SDF-1α can enhance this kind of interaction. To some extent, this finding confirmed that CXCR7 may heterodimerize with CXCR4 and regulate migration. Further studies using other methods such as bioluminescence resonance energy transfer (BRET), are still necessary to elucidate the specific interaction among SDF-1α, CXCR4 and CXCR7.
Previous studies have shown that β-arrestin1 plays important roles in migration, chemotaxis and invasion. β-arrestin1 regulates cell migration, and invasion in hepatocellular carcinoma cells and gastric cancer cells through the AKT/ERK signaling pathway (36, 37). Décaillot et al. (34) found that β-arrestin may be constitutively recruited by the CXCR7/CXCR4 heterodimer and participate in the enhancement of cell migration. To the best of our knowledge, the specific role of β-arrestin in PDLSC migration has not yet been reported. In the present study, we found that the expression of β-arrestin1 was promoted by SDF-1α stimulation in PDLSCs. After transfection with CXCR4 and CXCR7 siRNA, the expression of β-arrestin1 was suppressed. Moreover, in the PDLSCs transfected with ARRB1 siRNA, migration was suppressed, and p-ERK expression was decreased. Interestingly, we also found that treatment with an ERK inhibitor increased the expression of β-arre-stin1 in PDLSCs. This finding indicates that β-arrestin1 may be the upstream signaling molecule of the ERK signaling pathway, reacting to complex negative feedback with the ERK signaling pathway. The complicated interaction between β-arrestin1 and the ERK signaling pathway still needs further investigation.
Since root canal preparation and chemical disinfection cannot completely remove LPS from the root canal (20), we also investigated the influence of P. gingivalis LPS on PDLSC migration. We found that P. gingivalis LPS can promote the migration of PDLSCs toward SDF-1α by increasing the expression of CXCR4, promoting the expression of β-arrestin1, and then activating the ERK, and AKT signaling pathways through the NF-κB signaling pathway. Francis et al. (38) found that pretreatment of PDLSCs with LPS enhanced their migration, which was in accordance with our results. However, some researchers have reached the opposite conclusion. Yu et al. (39) found that 10 ng/ml P. gingivalis LPS suppressed the proliferation and migration of PDLSCs, in which a scratch assay was used to assess the migration and the concentration of LPS was far lower than that in our study. The difference in experimental conditions may be the reason for the different conclusions.
This study provides hints for further studies aiming to reveal the potential reason for the difficulty in forming pulp-dentin complexes, and provides theoretical guidance for the new clinical strategies, further promoting pulp-dentin complex regeneration. Our present study mainly focused on the mechanism of PDLSC migration. For further elucidation of the reason for the difficulty in realizing dentin pulp complex regeneration, further studies about the variation in migration between PDLSCs and SCAPs and the underlying mechanism are still necessary.
Notes
Supplementary Materials
Supplementary data including one figure can be found with this article online at https://doi.org/10.15283/ijsc22053.
References
1. Siqueira JF Jr, Rôças IN. Berman LH, Hargreaves KM, editors. 2016. Microbiology of endodontic infections. Cohen's Pathways of the Pulp. 11th ed. Elsevier;St. Louis: p. 599–629.
2. Murray PE, Garcia-Godoy F, Hargreaves KM. 2007; Regenerative endodontics: a review of current status and a call for action. J Endod. 33:377–390. DOI: 10.1016/j.joen.2006.09.013. PMID: 17368324.


3. Hargreaves KM, Diogenes A, Teixeira FB. 2013; Treatment options: biological basis of regenerative endodontic procedures. J Endod. 39(3 Suppl):S30–S43. DOI: 10.1016/j.joen.2012.11.025. PMID: 23439043. PMCID: PMC3589799.


4. Wang X, Thibodeau B, Trope M, Lin LM, Huang GT. 2010; Histologic characterization of regenerated tissues in canal space after the revitalization/revascularization procedure of immature dog teeth with apical periodontitis. J Endod. 36:56–63. DOI: 10.1016/j.joen.2009.09.039. PMID: 20003936.


5. Yamauchi N, Nagaoka H, Yamauchi S, Teixeira FB, Miguez P, Yamauchi M. 2011; Immunohistological characteri-zation of newly formed tissues after regenerative procedure in immature dog teeth. J Endod. 37:1636–1641. DOI: 10.1016/j.joen.2011.08.025. PMID: 22099896.


6. Martin G, Ricucci D, Gibbs JL, Lin LM. 2013; Histological findings of revascularized/revitalized immature permanent molar with apical periodontitis using platelet-rich plasma. J Endod. 39:138–144. DOI: 10.1016/j.joen.2012.09.015. PMID: 23228274.


7. Shimizu E, Ricucci D, Albert J, Alobaid AS, Gibbs JL, Huang GT, Lin LM. 2013; Clinical, radiographic, and histological observation of a human immature permanent tooth with chronic apical abscess after revitalization treatment. J Endod. 39:1078–1083. DOI: 10.1016/j.joen.2013.04.032. PMID: 23880282.


8. Altaii M, Richards L, Rossi-Fedele G. 2017; Histological assessment of regenerative endodontic treatment in animal studies with different scaffolds: a systematic review. Dent Traumatol. 33:235–244. DOI: 10.1111/edt.12338. PMID: 28342218.


9. Ducret M, Fabre H, Celle A, Mallein-Gerin F, Perrier-Groult E, Alliot-Licht B, Farges JC. 2017; Current challenges in human tooth revitalization. Biomed Mater Eng. 28(s1):S159–S168. DOI: 10.3233/BME-171637. PMID: 28372291.


10. Conde MC, Chisini LA, Demarco FF, Nör JE, Casagrande L, Tarquinio SB. 2016; Stem cell-based pulp tissue engineering: variables enrolled in translation from the bench to the bedside, a systematic review of literature. Int Endod J. 49:543–550. DOI: 10.1111/iej.12489. PMID: 26101143.


11. Kim JY, Xin X, Moioli EK, Chung J, Lee CH, Chen M, Fu SY, Koch PD, Mao JJ. 2010; Regeneration of dental-pulp-like tissue by chemotaxis-induced cell homing. Tissue Eng Part A. 16:3023–3031. DOI: 10.1089/ten.tea.2010.0181. PMID: 20486799. PMCID: PMC2947424.


12. Galler KM, Widbiller M. 2020; Cell-free approaches for dental pulp tissue engineering. J Endod. 46(9S):S143–S149. DOI: 10.1016/j.joen.2020.06.034. PMID: 32950186.


13. Xuan K, Li B, Guo H, Sun W, Kou X, He X, Zhang Y, Sun J, Liu A, Liao L, Liu S, Liu W, Hu C, Shi S, Jin Y. 2018; Deciduous autologous tooth stem cells regenerate dental pulp after implantation into injured teeth. Sci Transl Med. 10:eaaf3227. DOI: 10.1126/scitranslmed.aaf3227. PMID: 30135248.


14. Sui B, Chen C, Kou X, Li B, Xuan K, Shi S, Jin Y. 2019; Pulp stem cell-mediated functional pulp regeneration. J Dent Res. 98:27–35. DOI: 10.1177/0022034518808754. PMID: 30372659.


15. Marí-Beffa M, Segura-Egea JJ, Díaz-Cuenca A. 2017; Regene-rative endodontic procedures: a perspective from stem cell niche biology. J Endod. 43:52–62. DOI: 10.1016/j.joen.2016.09.011. PMID: 27986102.


16. Seo BM, Miura M, Gronthos S, Bartold PM, Batouli S, Brahim J, Young M, Robey PG, Wang CY, Shi S. 2004; Inves-tigation of multipotent postnatal stem cells from human periodontal ligament. Lancet. 364:149–155. DOI: 10.1016/S0140-6736(04)16627-0. PMID: 15246727.


17. Huang GT, Sonoyama W, Liu Y, Liu H, Wang S, Shi S. 2008; The hidden treasure in apical papilla: the potential role in pulp/dentin regeneration and bioroot engineering. J Endod. 34:645–651. DOI: 10.1016/j.joen.2008.03.001. PMID: 18498881. PMCID: PMC2653220.


18. Scadden DT. 2006; The stem-cell niche as an entity of action. Nature. 441:1075–1079. DOI: 10.1038/nature04957. PMID: 16810242.


19. Huo N, Tang L, Yang Z, Qian H, Wang Y, Han C, Gu Z, Duan Y, Jin Y. 2010; Differentiation of dermal multipotent cells into odontogenic lineage induced by embryonic and neonatal tooth germ cell-conditioned medium. Stem Cells Dev. 19:93–104. DOI: 10.1089/scd.2009.0048. PMID: 19469666.


20. Vianna ME, Horz HP, Gomes BP, Conrads G. 2006; In vivo evaluation of microbial reduction after chemo-mechanical pre-paration of human root canals containing necrotic pulp tissue. Int Endod J. 39:484–492. DOI: 10.1111/j.1365-2591.2006.01121.x. PMID: 16674744.


21. Feng YF, Guo H, Yuan F, Shen MQ. 2015; Lipopolysaccharide promotes choroidal neovascularization by up-regulation of CXCR4 and CXCR7 expression in choroid endothelial cell. PLoS One. 10:e0136175. DOI: 10.1371/journal.pone.0136175. PMID: 26288180. PMCID: PMC4545586. PMID: 1ba62d0e2d374f669476f951f54338aa.


22. Li N, Xu H, Ou Y, Feng Z, Zhang Q, Zhu Q, Cai Z. 2019; LPS-induced CXCR7 expression promotes gastric cancer proliferation and migration via the TLR4/MD-2 pathway. Diagn Pathol. 14:3. DOI: 10.1186/s13000-019-0780-x. PMID: 30636642. PMCID: PMC6330400. PMID: 0cb7cca0b67e499bbc5439908bcaa713.


23. Kukolj T, Trivanović D, Djordjević IO, Mojsilović S, Krstić J, Obradović H, Janković S, Santibanez JF, Jauković A, Bugarski D. 2018; Lipopolysaccharide can modify differentiation and immunomodulatory potential of periodontal ligament stem cells via ERK1,2 signaling. J Cell Physiol. 233:447–462. DOI: 10.1002/jcp.25904. PMID: 28295277.


24. Imitola J, Raddassi K, Park KI, Mueller FJ, Nieto M, Teng YD, Frenkel D, Li J, Sidman RL, Walsh CA, Snyder EY, Khoury SJ. 2004; Directed migration of neural stem cells to sites of CNS injury by the stromal cell-derived factor 1alpha/CXC chemokine receptor 4 pathway. Proc Natl Acad Sci U S A. 101:18117–18122. DOI: 10.1073/pnas.0408258102. PMID: 15608062. PMCID: PMC536055.


25. Naumann U, Cameroni E, Pruenster M, Mahabaleshwar H, Raz E, Zerwes HG, Rot A, Thelen M. 2010; CXCR7 functions as a scavenger for CXCL12 and CXCL11. PLoS One. 5:e9175. DOI: 10.1371/journal.pone.0009175. PMID: 20161793. PMCID: PMC2820091. PMID: 670aaf6339124a9a864e47a634a83cb5.


26. Feng YF, Yuan F, Guo H, Wu WZ. 2014; TGF-β1 enhances SDF-1-induced migration and tube formation of choroid-retinal endothelial cells by up-regulating CXCR4 and CXCR7 expression. Mol Cell Biochem. 397:131–138. DOI: 10.1007/s11010-014-2180-6. PMID: 25138701.


27. Levoye A, Balabanian K, Baleux F, Bachelerie F, Lagane B. 2009; CXCR7 heterodimerizes with CXCR4 and regulates CXCL12-mediated G protein signaling. Blood. 113:6085–6093. DOI: 10.1182/blood-2008-12-196618. PMID: 19380869.


28. Sierro F, Biben C, Martínez-Muñoz L, Mellado M, Ranso-hoff RM, Li M, Woehl B, Leung H, Groom J, Batten M, Harvey RP, Martínez-A C, Mackay CR, Mackay F. 2007; Disrupted cardiac development but normal hematopoiesis in mice deficient in the second CXCL12/SDF-1 receptor, CXCR7. Proc Natl Acad Sci U S A. 104:14759–14764. DOI: 10.1073/pnas.0702229104. PMID: 17804806. PMCID: PMC1976222.


29. Al-Habib M, Huang GT. 2019; Dental mesenchymal stem cells: dental pulp stem cells, periodontal ligament stem cells, apical papilla stem cells, and primary teeth stem cells-isolation, characterization, and expansion for tissue engineering. Methods Mol Biol. 1922:59–76. DOI: 10.1007/978-1-4939-9012-2_7. PMID: 30838565.


30. Gaudin A, Tolar M, Peters OA. 2018; Lipoxin A4 attenuates the inflammatory response in stem cells of the apical papilla via ALX/FPR2. Sci Rep. 8:8921. DOI: 10.1038/s41598-018-27194-7. PMID: 29892010. PMCID: PMC5995968.


31. Pizzatto LN, Meneses CCB, Diniz EA, Dionísio TJ, Santos CF, Sipert CR. 2020; Angiotensin II regulates proliferation and function of stem cells of apical papilla. J Endod. 46:810–817. DOI: 10.1016/j.joen.2020.03.015. PMID: 32331838.


32. Rubin JB. 2009; Chemokine signaling in cancer: one hump or two? Semin Cancer Biol. 19:116–122. DOI: 10.1016/j.semcancer.2008.10.001. PMID: 18992347. PMCID: PMC2694237.


33. Busillo JM, Benovic JL. 2007; Regulation of CXCR4 signaling. Biochim Biophys Acta. 1768:952–963. DOI: 10.1016/j.bbamem.2006.11.002. PMID: 17169327. PMCID: PMC1952230.


34. Décaillot FM, Kazmi MA, Lin Y, Ray-Saha S, Sakmar TP, Sachdev P. 2011; CXCR7/CXCR4 heterodimer constitutively recruits beta-arrestin to enhance cell migration. J Biol Chem. 286:32188–32197. DOI: 10.1074/jbc.M111.277038. PMID: 21730065. PMCID: PMC3173186.


35. Hernandez L, Magalhaes MA, Coniglio SJ, Condeelis JS, Segall JE. 2011; Opposing roles of CXCR4 and CXCR7 in breast cancer metastasis. Breast Cancer Res. 13:R128. DOI: 10.1186/bcr3074. PMID: 22152016. PMCID: PMC3326570.


36. Xu X, Lei Y, Zhou H, Guo Y, Liu H, Jiang J, Yang Y, Wu B. 2020; β-Arrestin1 is involved in hepatocellular carcinoma metastasis via extracellular signal-regulated kinase-mediated epithelial-mesenchymal transition. J Gastroenterol Hepatol. 35:2229–2240. DOI: 10.1111/jgh.15115. PMID: 32445259.


37. Xu T, Shen G, Cheng M, Wu X, Xu Y, Hu S. 2020; Upregulated β-arrestin1 predicts poor prognosis and promotes metastasis via AKT/ERK signaling pathway in gastric cancer. Pathol Res Pract. 216:153262. DOI: 10.1016/j.prp.2020.153262. PMID: 33129195.


38. Francis M, Pandya M, Gopinathan G, Lyu H, Ma W, Foyle D, Nares S, Luan X. 2019; Histone methylation mechanisms modulate the inflammatory response of periodontal ligament progenitors. Stem Cells Dev. 28:1015–1025. DOI: 10.1089/scd.2019.0125. PMID: 31218921. PMCID: PMC6661920.


39. Yu B, Li Q, Zhou M. 2019; LPS-induced upregulation of the TLR4 signaling pathway inhibits osteogenic differentiation of human periodontal ligament stem cells under inflam-matory conditions. Int J Mol Med. 43:2341–2351. DOI: 10.3892/ijmm.2019.4165.


Fig. 1
Migration of PDLSCs and SCAPs under normal and inflammation condition. (A∼E) SDF-1α improves the migration of PDLSCs and SCAPs in normal condition. The migrated PDLSCs cells were much more than SCAPs regardless of stimulated with SDF-1α or not. (F∼N) LPS improved the migration of PDLSCs and SCAPs in concentration-depended manner. The migrated PDLSCs cells were much more than SCAPs regardless of the LPS concentration. ****Indicates p<0.0001.
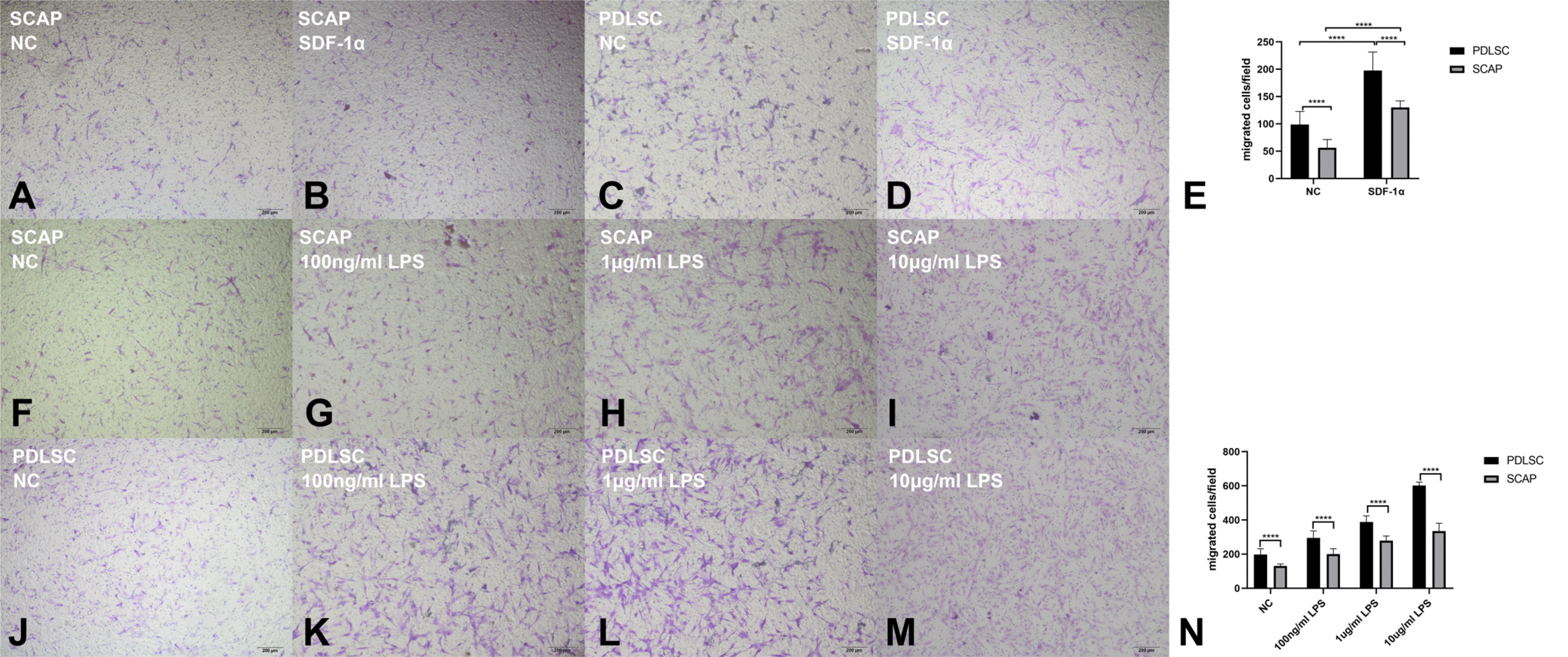
Fig. 2
CXCR4 and CXCR7 is related to migration of PDLSCs to SDF-1α. (A, B) qPCR assay revealed that SDF-1α increased the gene expression of CXCR4 and CXCR7 in PDLSCs. (C∼E) WB results revealed that that SDF-1α increases the expression of CXCR4 and CXCR7 protein. (F) CXCR4 interacts with CXCR7, which is improved by SDF-1α stimulation according to results of co-Immunoprecipitation assay. (G∼J) The siRNA of CXCR4 and CXCR7 can successfully suppress the expression of CXCR4 and CXCR7 according to WB results. (K∼N) Both siCXCR4 and siCXCR7 treatment suppress the migration of PDLSCs. *Indicates p<0.05, **Indicates p<0.01, ***Indicates p<0.001, ****Indicates p<0.0001.
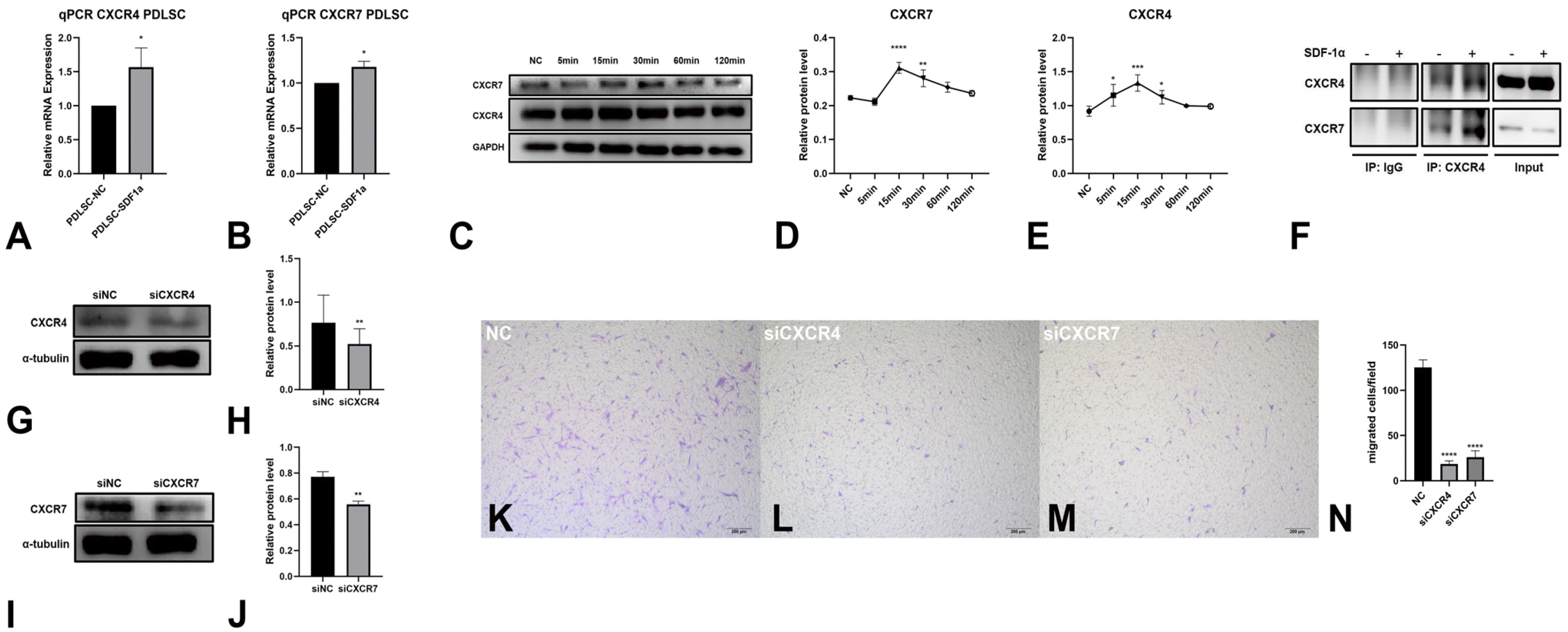
Fig. 3
ERK, AKT signal pathway and β-arrestin1 regulates the migration of PDLSCs towards SDF-1α. (A∼D) SDF-1α elevated the phos-phorylation level of ERK1/2 and AKT, as well as β-arrestin1. (Ea, b) The inhibitor of ERK can successfully suppress the expression of p-ERK. (Ia, b) The inhibitor of AKT can successfully suppress the expression of p-AKT. (Ma, b) The siRNA of ARRB1 can successfully suppress the expression of β-arrestin1 according to WB results. (F∼H, J∼L, N∼P) ERK inhibitor, AKT inhibitor treatment and siRNA of ARRB1 treatment suppressed the migration of PDLSCs. (Q∼T) SDF-1α activated the ERK and AKT signal pathway, improved the expression of β-arrestin1. Treatment of siRNA of CXCR4 and CXCR7 reversed this effect. (U∼W) Treatment of ERK inhibitor decreased the expression of p-ERK, increased the expression of β-arrestin1. (X, Y) Treatment of siRNA of β-arrestin1 decreased the expression of p-ERK. *Indicates p<0.05, **Indicates p<0.01, ***Indicates p<0.001, ****Indicates p<0.0001.
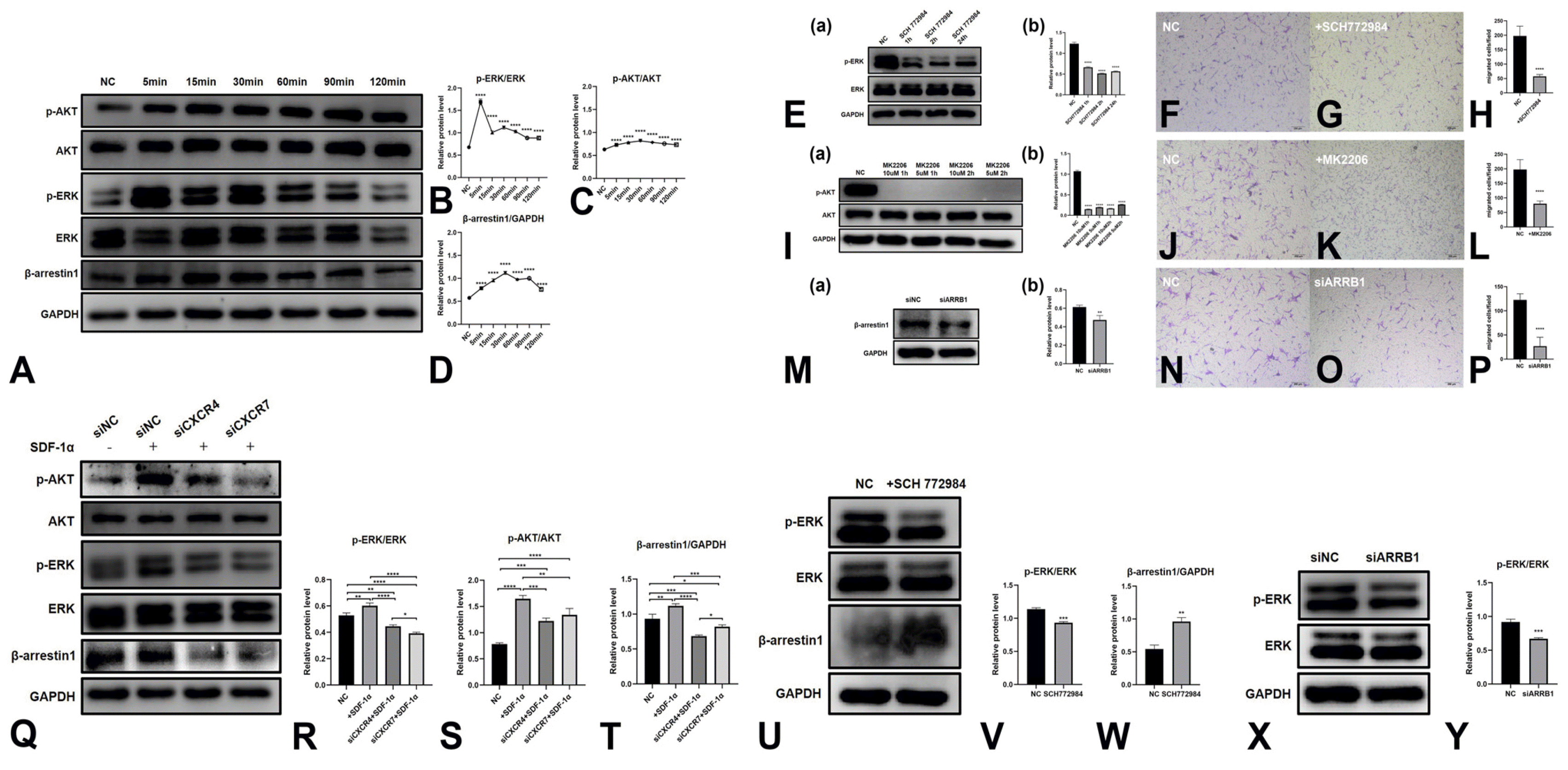
Fig. 4
CXCR4 expression is higher in inflammation condition. (Aa∼c) Representative images of apical lesion tissues and normal tissues (gingiva tissue and apical papilla) by hematoxylin and eosin staining. (Ad∼f) There was no positive expression of CXCR4 or CXCR7 in NC groups by immunohistochemistry staining. (Ag∼i) Much more fibroblast-like cells with positive expression of CXCR4 were presented in apical lesion tissue, comparing with normal gingiva tissue and apical papilla. (Aj∼l) CXCR7 were positively expressed in few cells in all tissues which mainly surround vessels. Almost no differences were shown in apical lesion tissues and normal tissues. (B, C) Expression of CXCR4 was higher in INFLAMMATION group while the expression of CXCR7 were nearly the same in two groups. (D, E) LPS treatment increased the CXCR4 mRNA expression in PDLSCs while almost did not affect the CXCR7 mRNA expression. (F∼I) LPS increased the CXCR4 protein expression in PDLSCs in time dependent pattern, while almost did not affect the CXCR7 protein expression. *Indicates p<0.05, ****Indicates p<0.0001.
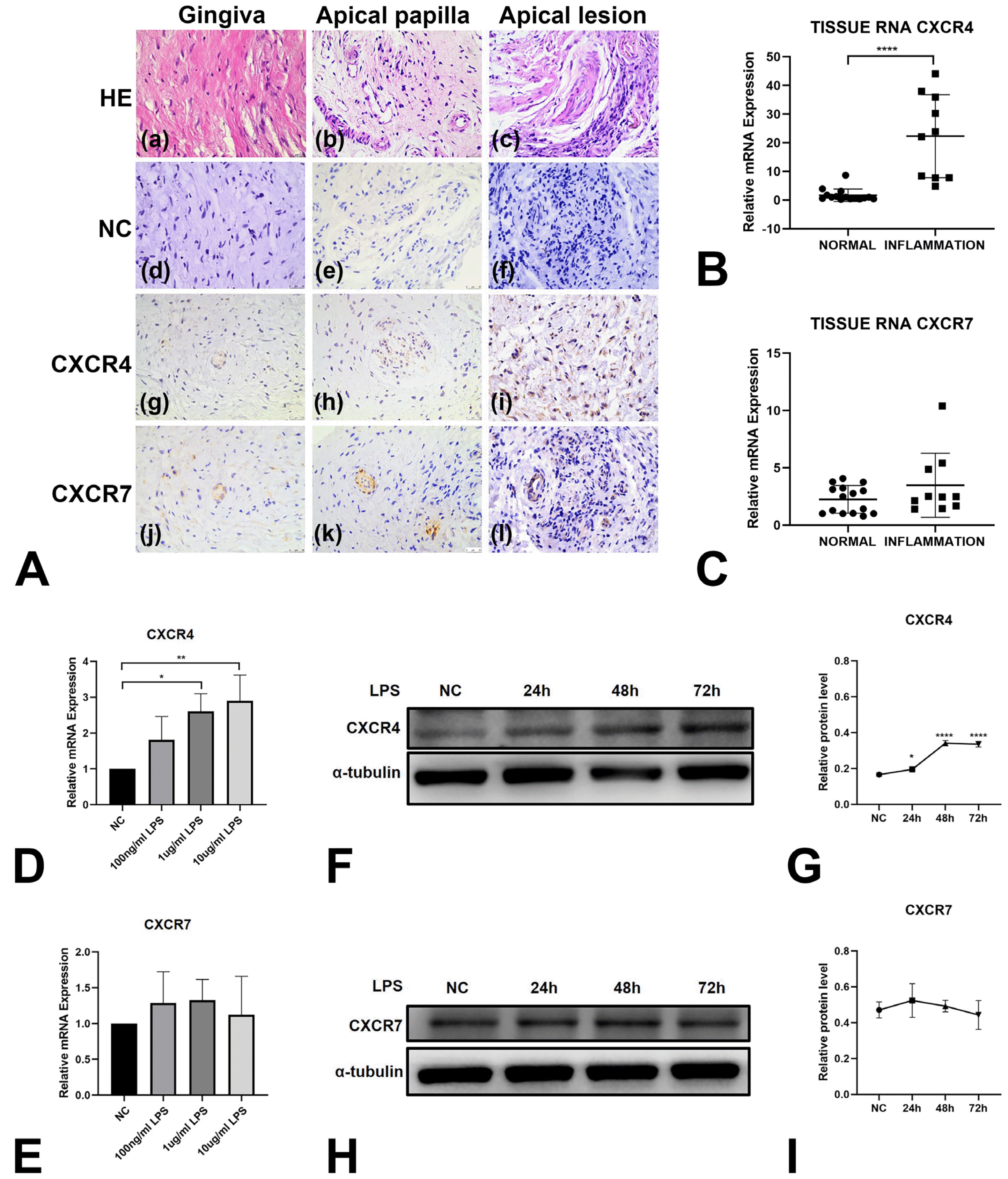
Fig. 5
P. gingivalis LPS can promote migration of PDLSC towards SDF-1α through increasing expression of CXCR4 by NF-κB signaling pathway, promoting expression of β-arrestin1, and then activating ERK signaling pathway. (A, B) LPS improved the p-P65 expression and activated the NF-κB signal pathway, which was reversed by NF-κB inhibitor treatment. (C∼F) LPS improved the migration of PDLSCs, which was reversed by NF-κB inhibitor treatment. (G∼I) LPS improved the CXCR4 expression and this effect was suppressed by NF-κB signal inhibitor treatment, while LPS and NF-κB signal inhibitor treatment almost did not affect the expression of CXCR7. (J∼M) LPS improved the expression of p-AKT, p-ERK and β-arrestin1. The NF-κB inhibitor treatment suppressed the effect of LPS. *Indicates p<0.05, **Indicates p<0.01, ***Indicates p<0.001, ****Indicates p<0.0001.
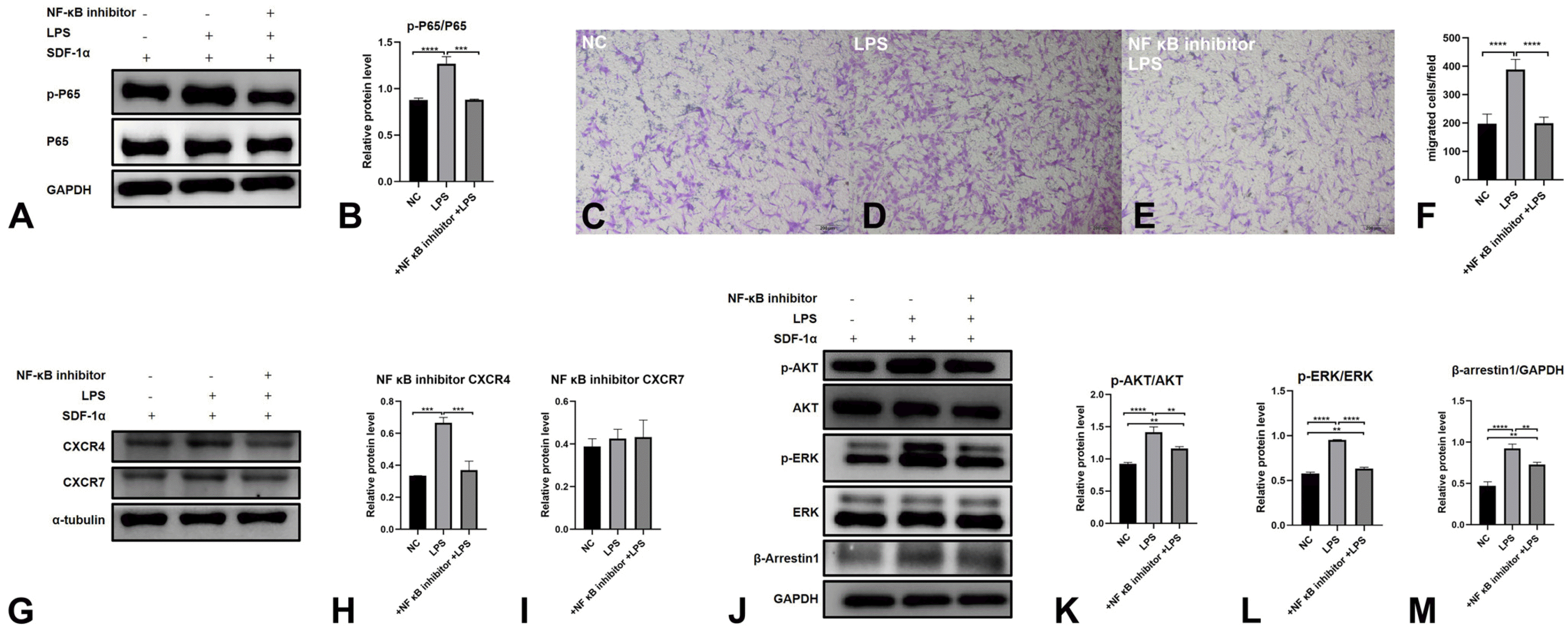
Table 1
The siRNA sequences used in siRNA transfection