Abstract
This study aimed to observe the protective effect of momordicine I, a triterpenoid compound extracted from momordica charantia L., on isoproterenol (ISO)-induced hypertrophy in rat H9c2 cardiomyocytes and investigate its potential mechanism. Treatment with 10 μM ISO induced cardiomyocyte hypertrophy as evidenced by increased cell surface area and protein content as well as pronounced upregulation of fetal genes including atrial natriuretic peptide, β-myosin heavy chain, and α-skeletal actin; however, those responses were markedly attenuated by treatment with 12.5 μg/ml momordicine I. Transcriptome experiment results showed that there were 381 and 447 differentially expressed genes expressed in comparisons of model/control and momordicine I intervention/model, respectively. GO enrichment analysis suggested that the anti-cardiomyocyte hypertrophic effect of momordicine I may be mainly associated with the regulation of metabolic processes. Based on our transcriptome experiment results as well as literature reports, we selected glycerophospholipid metabolizing enzymes group VI phospholipase A2 (PLA2G6) and diacylglycerol kinase ζ (DGK-ζ) as targets to further explore the potential mechanism through which momordicine I inhibited ISO-induced cardiomyocyte hypertrophy. Our results demonstrated that momordicine I inhibited ISO-induced upregulations of mRNA levels and protein expressions of PLA2G6 and DGK-ζ. Collectively, momordicine I alleviated ISO-induced cardiomyocyte hypertrophy, which may be related to its inhibition of the expression of glycerophospholipid metabolizing enzymes PLA2G6 and DGK-ζ.
Cardiac hypertrophy is a common pathological process in various cardiovascular diseases including coronary heart disease and hypertension, which eventually develops into heart failure and threatens human health. Currently, medications commonly used to treat cardiovascular diseases in the clinical such as angiotensin I converting enzyme inhibitor, angiotensin II receptor blocker, and β-receptor blocker, etc. can not completely block or reverse the development of cardiac hypertrophy. Previous studies have shown that abnormal lipid metabolism is one of the most important independent risk factors for cardiac hypertrophy and may affect the occurrence and development of cardiac hypertrophy [1]. When cardiac hypertrophy or diabetic heart disease occurred, the levels of some phospholipids and sphingolipids in the heart, for example, phosphatidylinositol, phosphatidylcholine and ceramide, were distinctly altered [2-4]. Recent studies found that some enzymes involved in glycerophospholipid metabolism were closely related to cardiac hypertrophy. A significant upregulation of CDP-diacylglycerol synthase 1 was observed in vasopressin-induced rat H9c2 cardiomyocyte hypertrophy model [5]. Diacylglycerol kinase ζ (DGK-ζ) was also upregulated in endothelin-1 induced neonatal rat cardiomyocytes hypertrophy model, whereas overexpression of DGK-ζ blocked endothelin-1 induced activation of PKCε-ERK-AP1 pathway, resulting in suppression of cardiac hypertrophy [6].
Momordica charantia L. is a kind of medicinal and edible plant that belongs to the Cucurbitaceae family. Literatures have shown that momordica charantia L. has multiple pharmacological properties such as anti-diabetic, anti-tumor, anti-lipolysis, anti-inflammatory, anti-bacterial, anti-oxidant, anti-ulcer, hepatoprotective, immunomodulation and deworming activities, and contains numerous phytochemicals such as saponins, polysaccharides, triterpenes, proteins, flavonoids, steroids and vitamins [7]. It is reported that polysaccharides, peptides, saponins and triterpenes can regulate glucose and lipid metabolism, and are considered to be the important functional components of momordica charantia L. [8]. Wen et al. [9] showed that combination of polysaccharides from both fermented and non-fermented momordica charantia L. with lactobacillus plantarum NCU116 improved glycerophospholipids, glycosphingolipids, and amino acid metabolism, thereby relieving hypercholesterolemia and overweight in obese rats. Momordica charantia insulin receptor binding peptid-19 (mcIRBP-19), one peptide from momordica charantia L. has been demonstrated to possess an insulin-like effect. A clinical study indicated that mcIRBP-19-containing momordica charantia extract can still reduce fasting blood glucose and hemoglobin A1c when anti-diabetic drugs are ineffective [10]. In addition, some saponins and triterpenoids from momordica charantia L. have been reported to stimulate insulin secretion and relieve hyperglycemia [11], particularly triterpenoids could also improve liver steatosis [12].
Momordicine I (Fig. 1A), a cucurbitane-type triterpenoid compound from stems, leaves and fruits of momordica charantia L., has been demonstrated to possess anti-tumor and anti-diabetic properties. A study reported that momordicine I can prevent the growth of head and neck tumors in mice by inhibiting c-Met and its downstream signaling [13]. Another study showed that momordicine I has inhibitory effects on high glucose-induced cell proliferation and collagen synthesis in rat cardiac fibroblasts, which may be related to nuclear factor erythroid 2-related factor 2-mediated inhibition of oxidative stress and transforming growth factor-beta 1 signaling [14]. However, it is unclear whether momordicine I exhibits anti-cardiac hypertrophy effects. In this study, we investigated the effect of momordicine I on isoproterenol (ISO)-induced cardiomyocyte hypertrophy. Furthermore, RNA sequencing, RT-PCR and Western blot were used to explore the possible molecular mechanism of momordicine I treatment for cardiac hypertrophy. The results of this study may provide an experimental basis for the application of momordicine I in the prevention and treatment of cardiovascular diseases.
Momordicine I was purchased from Shanghai Yuanye Bio-Technology Co., Ltd., purity > 95% (Shanghai, China). ISO was supplied by National Institutes for Food and Drug Control (Beijing, China). PrimeScript RT reagent Kit with gDNA Eraser (Perfect Real Time) and TB Green Premix Ex Taq II were obtained from Takara (Shiga, Japan). Anti-PLA2G6 (ab259950) and anti-DGK-ζ (ab239081) antibodies were provided by Abcam (Cambridge, UK). Anti-β-actin (sc-47778) was obtained from Santa Cruz Biotechnology Co., Ltd. (Dallas, TX, USA).
Rat H9c2 cardiomyocytes were provided by the Cell Resource Center, Peking Union Medical College (Beijing, China). Cells were maintained in Dulbecco’s modified Eagle’s medium (Solarbio, Beijing, China) supplemented with 10% fetal bovine serum (Gemini, Calabasas, CA, USA), and cultured in a humidified incubator at 37°C with 5% CO2. Cells were seeded in 35-mm culture dishes at a density of 5 × 103 per well (for measurement of cell surface area) or 1 × 105 per well (for measurements of protein content, RT-PCR, RNA sequencing and Western blot). After an overnight culture, cells were pretreated with momordicine I for 1 h followed by ISO treatment for 24 h. Based on previous study [15], 10 μM ISO was used to induce cardiomyocyte hypertrophy.
Effect of momordicine I on cardiomyocytes viability was determined by MTT assay. Cells were seeded in 96-well plates at a density of 1 × 104 per well, followed by treatment with different concentrations of momordicine I (1.625, 3.125, 6.25, 12.5, 25.0 μg/ml) for 24 h. Afterwards, 50 μl MTT solution (1 mg/ml) was added to each well for 4 h. The medium was discarded and 150 μl dimethylsulfoxide was added to each well. The absorbance was measured with a microplate reader (Molecular Devices, San Jose, CA, USA) at 490 nm.
The images of cardiomyocytes were visualized using MI52-N inverted microscope (Guangzhou Mingmei Photoelectric Technology Co., Ltd., Guangzhou, China) equipped with MShot Image Analysis System at 100× magnification. The surface area of a minimum of 50 cells per treatment group was measured by ImageJ software and averaged to produce one N value.
Cells were separated by trypsin and counted, then washed three times with cold phosphate buffered saline. Cells were lysed with 0.5% Triton X-100 for 30 min at 4°C. The protein content of the lysate was determined with a BCA protein assay kit (Beyotime, Shanghai, China), and then the protein concentration per 105 cells was calculated. The experiments were repeated 3 times.
Total RNA was extracted from cardiomyocytes with TRIzol reagent according to the manufacturer’s instructions and quantified by measuring the absorbance at 260 nm. 1 μg of total RNA was reverse transcribed into cDNA using the PrimeScript RT reagent kit at 37˚C for 15 min and then 85˚C for 5 sec. PCR amplification was conducted with the TB Green Premix Ex Taq II using CFX96 Real-Time PCR (Bio-Rad, Hercules, CA, USA). The PCR conditions were pre-denaturation at 95°C for 30 sec, followed by 40 cycles of 95°C for 10 sec (denaturation), 60°C for 30 sec (annealing), and 72°C for 30 sec (extension). The mRNA relative expression of atrial natriuretic peptide (ANP), β-myosin heavy chain (β-MHC), and α-skeletal actin (α-SKA) was calculated according to the standard curve with 18S rRNA as a loading internal control. Primer sequences were shown in Table 1.
Cardiomyocytes were harvested to extract RNA according to the manufacturer’s instructions. Transcriptome sequencing of RNA was completed by Biomarker Technologies (Beijing, China) as described previously [16]. In brief, the purity and integrity of RNA were detected by NanoDrop ND-1000 spectrophotometer (Thermo Scientific, Wilmington, DE, USA) and Bioanalyzer 2100 (Agilent Technologies, Palo Alto, CA, USA), and the cDNA library was constructed using the equal quantities of RNA of three individual samples in each group and sequenced on the Illumina sequencing platform (NovaSeq 6000). The sequenced data was filtered to obtain high quality data (clean data), and the sequences were compared with the specified reference genome to obtain the Mapped Data. The differentially expressed genes (DEGs) in the samples were defined with DEGseq (|Fold Change| ≥ 1.5 and FDR < 0.05) by the DESeq2 software. Finally, GO enrichment analysis of DEGs was performed using the R package.
In order to validate the results of the transcriptome analyses, the mRNA and protein levels of group VI phospholipase A2 (PLA2G6) and DGK-ζ, two enzymes involved in glycerophospholipid metabolism pathway, were determined by RT-PCR and Western blot, respectively. The method of RT-PCR was the same as described above, and the primer sequences were also listed in Table 1. The method of Western blot was briefly described as follows. Cardiomyocytes sample was lysed with radioimmunoprecipitation assay buffer containing 1 mM phenylmethylsulphonyl fluoride and protein concentration was estimated by BCA reagent. 40 μg protein was separated by 10% SDS-PAGE, transferred to PVDF membrane, and blocked in 5% bovine serum albumin at room temperature for 1 h. Subsequently, the membrane was incubated with primary antibody anti-PLA2G6 (1:2,000 dilution), anti-DGK-ζ (1:2,000 dilution) and anti-β-actin (1:500 dilution) at 4°C overnight, respectively, then incubated with secondary antibody at room temperature for 30 min. Finally, the membrane was detected by ECL detection kit and the protein band was semiquantitatively analyzed by ImageJ software.
As shown in Fig. 1B, compared with the control group, no significant differences were observed in cell viability when cardiomyocytes were exposed to momordicine I in the concentration range of 1.625–12.5 μg/ml (p > 0.05), however, a significant drop in cell viability was seen when cardiomyocytes were treated with momordicine I 25 μg/ml (p < 0.05). Therefore, 12.5 μg/ml of momordicine I was used in the subsequent experiments in this study.
To investigate the inhibitory effect of momordicine I on ISO-induced cardiomyocyte hypertrophy, we measured cardiomyocyte surface area, protein content and the mRNA expression of fetal genes. As shown in Fig. 2, compared with the control group, 10 μM ISO treatment resulted in cardiomyocyte hypertrophy as evidenced by increased cell surface area and protein content as well as significant upregulation of fetal genes including ANP, β-MHC, and α-SKA (p < 0.05), however, those responses were markedly attenuated by treatment with 12.5 μg/ml momordicine I (p < 0.05). All these results clearly demonstrated that momordicine I could inhibit ISO-induced cardiomyocyte hypertrophy.
To investigate the gene expression changes of momordicine I in alleviating ISO-induced cardiomyocyte hypertrophy, gene transcriptional analysis was performed in RNA samples of cardiomyocytes. Statistically significant DEGs (|Fold Change| ≥ 1.5 and FDR < 0.05) were screened by DESeq2 software. Compared with the control group, 202 genes were upregulated and 179 genes were downregulated in the ISO group, whereas 301 genes were upregulated and 298 genes were downregulated in momordicine I alone group. Compared with the ISO group, 237 genes were upregulated and 210 genes were downregulated after treatment with 12.5 μg/ml momordicine I (Fig. 3A). Volcano plots of DEGs between model/control, momordicine I intervention/model, and momordicine I alone/control comparisons were shown in Fig. 3B–D, respectively.
To further explore the biological effects that momordicine I regulates ISO-induced cardiomyocyte hypertrophy, GO enrichment analysis was used to conduct the functional classification of the identified DEGs. All DEGs were annotated to the same functional GO categories between the three comparisons. The results showed that it includes 21 biological processes, 18 cellular components, and 17 molecular functions, with 56 functional categories in total. The top 10 items for each functional category were listed in Table 2. In the “biological processes” category, DEGs were primarily involved in the regulation of “cellular process” and “metabolic process”. There were 230 and 293 DEGs expressed in comparisons of model/control and momordicine I intervention/model of “cellular process”, respectively, whereas in the “metabolic process”, there were 185 and 274 DEGs, respectively. Moreover, the highest numbers of DEGs were enriched in the items of “cell” and “cell part” in the “cellular components” category, and items of “binding” and “catalytic activity” in the “molecular function” category. The results of GO enrichment analysis suggest that the anti-cardiomyocyte hypertrophic effect of momordicine I may be mainly associated with the regulation of metabolic processes.
Literatures showed that glycerophospholipid metabolizing enzymes both PLA2G6 and DGK-ζ are closely related to cardiac hypertrophy [17,18]. According to our results of transcriptome experiments, we selected PLA2G6 and DGK-ζ as targets to further explore the role of momordicine I on the regulation of metabolic processes in ISO-induced cardiomyocyte hypertrophy. As shown in Fig. 4, the mRNA levels and protein expressions of PLA2G6 and DGK-ζ in the ISO group were significantly upregulated than those in the control group (p < 0.05), although these increases were significantly blunted by treatment with 12.5 μg/ml momordicine I (p < 0.05).
The major finding of this study is that momordicine I effectively inhibited ISO-induced cardiomyocyte hypertrophy as evidenced by reductions in cell surface area and protein content, as well as downregulation of hypertrophy-related fetal gene expression in cardiomyocytes. These effects were further demonstrated to be associated with suppression of the expressions of PLA2G6 and DGK-ζ, suggesting the potential efficacy of momordicine I in cardiac hypertrophy by targeting glycerophospholipid metabolizing enzymes.
Cardiac hypertrophy is a response of myocardium to various stimuli such as ischemia and hypoxia, pressure or volume overload, hormones, etc. It is characterized by increases in cardiomyocyte size and protein synthesis as well as reactivation of fetal genes related to cardiac hypertrophy [19]. Isoproterenol, a synthetic catecholamine and β-adrenergic agonist, is primarily used in the treatment of bradycardia, heart atrioventricular block and bronchial asthma. However, excessive or long-term use of isoproterenol is known to cause an increase in myocardial oxygen consumption, which results in enhanced cardiac load and circulatory dysfunction. Therefore, isoproterenol has been widely used to induce cardiac hypertrophy and heart failure models in experimental animals [20].
Momordica charantia L. was reported to serve as a potential adjuvant therapy for cardiovascular disorders due to its effects of improvement of cardiac function, suppression of post-ischemic/reperfused infarct size and modulation of serum cholesterol [21]. Cui et al. [22] found that momordica charantia L.-derived extracellular vesicles-like nanovesicles protected cardiomyocytes against radiation injury by alleviating DNA damage and mitochondria dysfunction. Additionally, momordica charantia extract inhibited endogenous conversion of squalene to cholesterol in cardiac tissue thereby reducing cholesterol level in breast cancer rats [23], and also improved redox imbalance associated with cardiac dysfunction in doxorubicin stressed rats [24].
Cucurbitane-type triterpenoids are a major class of active ingredients present in momordica charantia L., although there are few studies on its pharmacological effects and molecular mechanisms. Momordicine I, a cucurbitane triterpenoid with relatively high content in momordica charantia L. [25], has been reported to exhibit multiple activities, including anti-tumor [13], anti-diabetic [14] and deworming [26], and so on. In this study, we demonstrated for the first time that 12.5 μg/ml momordicine I exerts anti-cardiomyocyte hypertrophic effect, effectively inhibiting ISO-induced increases in cell surface area and protein content, as well as upregulation of hypertrophy-related fetal genes including ANP, β-MHC, and α-SKA in cardiomyocytes. To understand potential mechanisms by which momordicine I inhibits ISO-induced hypertrophic effect, we used RNA sequencing to measure the differential gene expression in cardiomyocytes. Our results showed that 381 and 447 DEGs were found between model/normal and momordicine I intervention/model comparisons, respectively. GO enrichment analysis indicated the highest numbers of DEGs were enriched in the items of “cellular process”, “metabolic process”, “cell”, “cell part”, “binding” and “catalytic activity” in the three categories (biological process, cellular component, and molecular function). We deduced that the anti-cardiomyocyte hypertrophic effect of momordicine I may be primarily related to the regulation of metabolic processes.
It is well known that alterations in metabolic process especially lipid metabolism, for example phospholipid metabolism or sphingolipid metabolism, is an intergrated part of metabolic and structural remodeling in the development of cardiac hypertrophy [27]. Phospholipids are the principal components of cell membranes and organelles. They possess biological activities and participate in many important physiopathological processes such as cell growth, differentiation, proliferation, migration, metabolism and death, etc. [28]. A clinical study revealed that phospholipids especially phosphatidylcholines are closely related to the occurrence of coronary heart disease, which illustrates that glycerophospholipid metabolic pathway is one of the main pathways of coronary heart disease lipid metabolism disorders [29]. Moreover, the paralleling results were obtained in another case-cohort study, the results of which showed that serum metabolites diacyl-phosphatidylcholine C38:3, C40:4 and acyl-alkylphosphatidylcholine C36:3 are associated with the risk of myocardial infarction [30]. Animal experimental studies have shown that alterations in cardiolipin, phosphatidylcholine and lysophosphatidic acid were observed when myocardial infarction or reperfusion injury occurred. Cardiolipin, a mitochondria-specific phospholipid, is actively involved in multiple processes of mitochondrial bioenergetics, dynamics, mitophagy and apoptosis. It is well known that alterations in cardiolipin may negatively impact mitochondrial function, with important implications in a variety of pathophysiological situations including cardiomyocyte injury [31]. Stamenkovic et al. [32] found myocardial ischemia-reperfusion injury increases the generation of oxidized phosphatidylcholines, which disrupted mitochondrial bioenergetics and calcium transients, and induced ferroptosis in cardiomyocytes. Wang et al. [33] indicated lysophosphatidic acid receptor 3 (LPA3)-mediated LPA signaling plays an important role for cardiomyocyte proliferation in the early postnatal heart. Cardiac-specific LPA3 overexpression improved cardiac function and promoted cardiac regeneration after myocardial infarction.
It was reported that some glycerophospholipid metabolizing enzymes including cytosolic calcium-independent phospholipase A2-β (iPLA2-β, PLA2G6), diacylglycerol kinases (DGKs), phosphatidic acid phosphatase (lipin) and cardiolipin synthase (CRLS), are closely associated with the occurrence and development of cardiac hypertrophy. Calcium-independent phospholipase A2 (iPLA2) is the predominant phospholipase A2 present in heart, which can hydrolyze membrane phospholipid to generate arachidonic acid and lysophosphatidylcholine. A literature showed that PLA2G6 is one of the risk genes for ischemic or hemorrhagic stroke [34]. The expression of iPLA2-β is upregulated following myocardial ischemia-reperfusion injury, and increased iPLA2-β translocates to endoplasmic reticulum (ER), thus resulting in ER stress-induced cardiomyocyte apoptosis [17]. Besides, the activity of iPLA2-γ is also increased in failing human myocardium, and activation of iPLA2-γ leads to production of toxic hydroxyeicosatetraenoic acids, which promotes the opening of mitochondrial permeability transition pore resulting in mitochondrial dysfunction and aggravation of heart failure [35]. DGKs are a family of enzymes that catalyze the conversion of diacylglycerol into phosphatidic acid. So far, DGKs have at least 10 subtypes in mammalian, of which DGK-ζ, ε, and η are all expressed in the heart [36]. Studies have shown that level of DGK-ζ mRNA is enhanced in an in vivo rat model of myocardial infarction [18] and an in vitro model of neonatal rat cardiomyocyte hypertrophy [5]. The expression of DGK-ζ is increased in the infarcted and border areas in the early stage of myocardial infarction in rats, and that may be attributed to granulocytes and macrophages [18]. Moreover, in the mouse models of juvenile arthritis and cytokine storm syndrome, DGK-ζ could regulate macrophage-mediated inflammatory responses through DGK-ζ-DAG/phosphatidic acid axis [37]. Additionally, studies have shown that lipin1 is downregulated in failing human heart, and cardiac specific lipin1 knockout resulted in a mild cardiac hypertrophy and impaired cardiac functional reserve in mice [38]. Moreover, lipin1 is also considered as a new target gene for essential hypertension [39]. CRLS, a crucial enzyme for the biosynthesis of mitochondrial cardiolipin, is downregulated in cardiomyocytes following catecholamine-induced cardiac damage in mice, accompanied by increased oxygen consumption rates, signs of oxidative stress and mitochondrial uncoupling [40].
According to our transcriptome experiment results as well as literature reports mentioned above, we selected the enzymes PLA2G6 and DGK-ζ as targets, to further explore whether the anti-cardiomyocyte hypertrophy effect of momordicine I is associated with glycerophospholipid metabolizing enzymes. Our results showed that the mRNA levels and protein expressions of PLA2G6 and DGK-ζ are significantly upregulated in ISO-induced cardiomyocyte hypertrophy model, which is consistent with the literatures [5,17,18]. These effects were reduced by momordicine I treatment.
In summary, our study firstly demonstrates that momordicine I inhibits ISO-induced cardiomyocyte hypertrophy. Moreover, we further show that the mechanism of this effect is related to suppression of the expressions of PLA2G6 and DGK-ζ. Therefore, momordicine I may be an effective therapy to prevent and treat cardiovascular diseases by targeting glycerophospholipid metabolic pathway.
Notes
FUNDING
This work was supported by the grants from the National Natural Science Foundation of China (Nos.81960732, 82060733), Natural Science Foundation of Jiangxi Province (2018BAB215041), Startup Foundation for Doctors of Jiangxi Science & Technology Normal University (Nos.2017BSQD017), Open Project of Jiangxi Provincial Key Laboratory of Drug Design and Evaluation (JKLDE-KF-2101), and Open Project of Key Laboratory of Modern Preparation of TCM, Ministry of Education, Jiangxi University of Chinese Medicine (TCM-201911).
REFERENCES
1. Sagris M, Antonopoulos AS, Theofilis P, Oikonomou E, Siasos G, Tsalamandris S, Antoniades C, Brilakis ES, Kaski JC, Tousoulis D. 2022; Risk factors profile of young and older patients with myocardial infarction. Cardiovasc Res. 118:2281–2292. DOI: 10.1093/cvr/cvab264. PMID: 34358302. PMID: https://www.scopus.com/inward/record.uri?partnerID=HzOxMe3b&scp=85135382443&origin=inward.
2. Tham YK, Bernardo BC, Huynh K, Ooi JYY, Gao XM, Kiriazis H, Giles C, Meikle PJ, McMullen JR. 2018; Lipidomic profiles of the heart and circulation in response to exercise versus cardiac pathology: a resource of potential biomarkers and drug targets. Cell Rep. 24:2757–2772. DOI: 10.1016/j.celrep.2018.08.017. PMID: 30184508. PMID: https://www.scopus.com/inward/record.uri?partnerID=HzOxMe3b&scp=85052909594&origin=inward.
3. David CEB, Lucas AMB, Cunha PLO, Viana YIP, Yoshinaga MY, Miyamoto S, Filho ABC, Varela ALN, Kowaltowski AJ, Facundo HT. 2022; Calorie restriction changes lipidomic profiles and maintains mitochondrial function and redox balance during isoproterenol-induced cardiac hypertrophy. J Physiol Biochem. 78:283–294. DOI: 10.1007/s13105-021-00863-4. PMID: 35023023. PMID: https://www.scopus.com/inward/record.uri?partnerID=HzOxMe3b&scp=85122782360&origin=inward.
4. Dong S, Zhang R, Liang Y, Shi J, Li J, Shang F, Mao X, Sun J. 2017; Changes of myocardial lipidomics profiling in a rat model of diabetic cardiomyopathy using UPLC/Q-TOF/MS analysis. Diabetol Metab Syndr. 9:56. DOI: 10.1186/s13098-017-0249-6. PMID: 28736579. PMCID: PMC5520292. PMID: https://www.scopus.com/inward/record.uri?partnerID=HzOxMe3b&scp=85025078604&origin=inward.
5. Blunsom NJ, Gomez-Espinosa E, Ashlin TG, Cockcroft S. 2019; Sustained phospholipase C stimulation of H9c2 cardiomyoblasts by vasopressin induces an increase in CDP-diacylglycerol synthase 1 (CDS1) through protein kinase C and cFos. Biochim Biophys Acta Mol Cell Biol Lipids. 1864:1072–1082. DOI: 10.1016/j.bbalip.2019.03.002. PMID: 30862571. PMCID: PMC6495107. PMID: https://www.scopus.com/inward/record.uri?partnerID=HzOxMe3b&scp=85062812354&origin=inward.
6. Takahashi H, Takeishi Y, Seidler T, Arimoto T, Akiyama H, Hozumi Y, Koyama Y, Shishido T, Tsunoda Y, Niizeki T, Nozaki N, Abe J, Hasenfuss G, Goto K, Kubota I. 2005; Adenovirus-mediated overexpression of diacylglycerol kinase-zeta inhibits endothelin-1-induced cardiomyocyte hypertrophy. Circulation. 111:1510–1516. DOI: 10.1161/01.CIR.0000159339.00703.22. PMID: 15781737. PMID: https://www.scopus.com/inward/record.uri?partnerID=HzOxMe3b&scp=20144389232&origin=inward.
7. Jia S, Shen M, Zhang F, Xie J. 2017; Recent advances in Momordica charantia: functional components and biological activities. Int J Mol Sci. 18:2555. DOI: 10.3390/ijms18122555. PMID: 29182587. PMCID: PMC5751158. PMID: https://www.scopus.com/inward/record.uri?partnerID=HzOxMe3b&scp=85036544468&origin=inward.
8. Liu Z, Gong J, Huang W, Lu F, Dong H. 2021; The effect of Momordica charantia in the treatment of diabetes mellitus: a review. Evid Based Complement Alternat Med. 2021:3796265. DOI: 10.1155/2021/3796265. PMID: 33510802. PMCID: PMC7826218. PMID: https://www.scopus.com/inward/record.uri?partnerID=HzOxMe3b&scp=85099903765&origin=inward.
9. Wen JJ, Li MZ, Gao H, Hu JL, Nie QX, Chen HH, Zhang YL, Xie MY, Nie SP. 2021; Polysaccharides from fermented Momordica charantia L. with Lactobacillus plantarum NCU116 ameliorate metabolic disorders and gut microbiota change in obese rats. Food Funct. 12:2617–2630. DOI: 10.1039/D0FO02600J. PMID: 33634806. PMID: https://www.scopus.com/inward/record.uri?partnerID=HzOxMe3b&scp=85103480854&origin=inward.
10. Yang YS, Wu NY, Kornelius E, Huang CN, Yang NC. 2022; A randomized, double-blind, placebo-controlled trial to evaluate the hypoglycemic efficacy of the mcIRBP-19-containing Momordica charantia L. fruit extracts in the type 2 diabetic subjects. Food Nutr Res. 66:3685. DOI: 10.29219/fnr.v66.3685. PMID: 35140559. PMCID: PMC8788657. PMID: c80452100f314fab90d29cc70b725d16. PMID: https://www.scopus.com/inward/record.uri?partnerID=HzOxMe3b&scp=85128399102&origin=inward.
11. Keller AC, Ma J, Kavalier A, He K, Brillantes AM, Kennelly EJ. 2011; Saponins from the traditional medicinal plant Momordica charantia stimulate insulin secretion in vitro. Phytomedicine. 19:32–37. DOI: 10.1016/j.phymed.2011.06.019. PMID: 22133295. PMCID: PMC3389550. PMID: https://www.scopus.com/inward/record.uri?partnerID=HzOxMe3b&scp=82755189591&origin=inward.
12. Dwijayanti DR, Shimada T, Ishii T, Okuyama T, Ikeya Y, Mukai E, Nishizawa M. 2020; Bitter melon fruit extract has a hypoglycemic effect and reduces hepatic lipid accumulation in ob/ob mice. Phytother Res. 34:1338–1346. DOI: 10.1002/ptr.6600. PMID: 31845444. PMID: https://www.scopus.com/inward/record.uri?partnerID=HzOxMe3b&scp=85076766699&origin=inward.
13. Sur S, Steele R, Isbell TS, Venkata KN, Rateb ME, Ray RB. 2021; Momordicine-I, a bitter melon bioactive metabolite, displays anti-tumor activity in head and neck cancer involving c-met and downstream signaling. Cancers (Basel). 13:1432. DOI: 10.3390/cancers13061432. PMID: 33801016. PMCID: PMC8003975. PMID: 85f8afb5522743ea8bb4741fc9052940. PMID: https://www.scopus.com/inward/record.uri?partnerID=HzOxMe3b&scp=85102685622&origin=inward.
14. Chen PY, Shih NL, Hao WR, Chen CC, Liu JC, Sung LC. 2018; Inhibitory effects of momordicine I on high-glucose-induced cell proliferation and collagen synthesis in rat cardiac fibroblasts. Oxid Med Cell Longev. 2018:3939714. DOI: 10.1155/2018/3939714. PMID: 30402205. PMCID: PMC6196925. PMID: https://www.scopus.com/inward/record.uri?partnerID=HzOxMe3b&scp=85056253666&origin=inward.
15. Shang L, Pin L, Zhu S, Zhong X, Zhang Y, Shun M, Liu Y, Hou M. 2019; Plantamajoside attenuates isoproterenol-induced cardiac hypertrophy associated with the HDAC2 and AKT/GSK-3β signaling pathway. Chem Biol Interact. 307:21–28. DOI: 10.1016/j.cbi.2019.04.024. PMID: 31009642. PMID: https://www.scopus.com/inward/record.uri?partnerID=HzOxMe3b&scp=85064639704&origin=inward.
16. Gao S, Zhang Q, Tian C, Li C, Lin Y, Gao W, Wu D, Jiao N, Zhu L, Li W, Zhu R, Wang W, Wang Y. 2020; The roles of Qishen granules recipes, Qingre Jiedu, Wenyang Yiqi and Huo Xue, in the treatment of heart failure. J Ethnopharmacol. 249:112372. DOI: 10.1016/j.jep.2019.112372. PMID: 31683036. PMID: https://www.scopus.com/inward/record.uri?partnerID=HzOxMe3b&scp=85075378732&origin=inward.
17. Jin T, Lin J, Gong Y, Bi X, Hu S, Lv Q, Chen J, Li X, Chen J, Zhang W, Wang M, Fu G. 2021; iPLA2β contributes to ER stress-induced apoptosis during myocardial ischemia/reperfusion injury. Cells. 10:1446. DOI: 10.3390/cells10061446. PMID: 34207793. PMCID: PMC8227999. PMID: 023c908438c945ffb7bf1b6d77c13e8c. PMID: https://www.scopus.com/inward/record.uri?partnerID=HzOxMe3b&scp=85110305695&origin=inward.
18. Takeda M, Kagaya Y, Takahashi J, Sugie T, Ohta J, Watanabe J, Shirato K, Kondo H, Goto K. 2001; Gene expression and in situ localization of diacylglycerol kinase isozymes in normal and infarcted rat hearts: effects of captopril treatment. Circ Res. 89:265–272. DOI: 10.1161/hh1501.094185. PMID: 11485977. PMID: https://www.scopus.com/inward/record.uri?partnerID=HzOxMe3b&scp=0035800890&origin=inward.
19. Nakamura M, Sadoshima J. 2018; Mechanisms of physiological and pathological cardiac hypertrophy. Nat Rev Cardiol. 15:387–407. DOI: 10.1038/s41569-018-0007-y. PMID: 29674714. PMID: https://www.scopus.com/inward/record.uri?partnerID=HzOxMe3b&scp=85045747976&origin=inward.
20. Liu Y, Chen J, Fontes SK, Bautista EN, Cheng Z. 2022; Physiological and pathological roles of protein kinase A in the heart. Cardiovasc Res. 118:386–398. DOI: 10.1093/cvr/cvab008. PMID: 33483740. PMCID: PMC8803072. PMID: https://www.scopus.com/inward/record.uri?partnerID=HzOxMe3b&scp=85123969706&origin=inward.
21. Czompa A, Gyongyosi A, Szoke K, Bak I, Csepanyi E, Haines DD, Tosaki A, Lekli I. 2017; Effects of Momordica charantia (bitter melon) on ischemic diabetic myocardium. Molecules. 22:488. DOI: 10.3390/molecules22030488. PMID: 28335529. PMCID: PMC6155383. PMID: https://www.scopus.com/inward/record.uri?partnerID=HzOxMe3b&scp=85016285017&origin=inward.
22. Cui WW, Ye C, Wang KX, Yang X, Zhu PY, Hu K, Lan T, Huang LY, Wang W, Gu B, Yan C, Ma P, Qi SH, Luo L. 2022; Momordica. charantia-derived extracellular vesicles-like nanovesicles protect cardiomyocytes against radiation injury via attenuating DNA damage and mitochondria dysfunction. Front Cardiovasc Med. 9:864188. DOI: 10.3389/fcvm.2022.864188. PMID: 35509278. PMCID: PMC9058095. PMID: 20f40213d7f1470baaccdffc8eb74cd9. PMID: https://www.scopus.com/inward/record.uri?partnerID=HzOxMe3b&scp=85138659076&origin=inward.
23. Białek A, Białek M, Lepionka T, Pachniewicz P, Czauderna M. 2021; Oxysterols and lipidomic profile of myocardium of rats supplemented with pomegranate seed oil and/or bitter melon aqueous extract - cardio-oncological animal model research. Chem Phys Lipids. 235:105057. DOI: 10.1016/j.chemphyslip.2021.105057. PMID: 33515592. PMID: https://www.scopus.com/inward/record.uri?partnerID=HzOxMe3b&scp=85100404502&origin=inward.
24. Saliu JA, Oyeleye SI, Olasehinde TA, Oboh G. 2022; Modulatory effects of stonebreaker (Phyllanthus amarus) and bitter gourd (Momordica charantia) on enzymes linked with cardiac function in heart tissue of doxorubicin-stressed rats. Drug Chem Toxicol. 45:331–339. DOI: 10.1080/01480545.2019.1700271. PMID: 31823659. PMID: https://www.scopus.com/inward/record.uri?partnerID=HzOxMe3b&scp=85076399953&origin=inward.
25. Lee YT, Pao LH, Chen CY, Huang SQ, Kumaran A, Chyuan JH, Chiu CH. 2022; Microwave- and ultrasound-assisted extraction of cucurbitane-type triterpenoids from Momordica charantia L. cultivars and their antiproliferative effect on SAS human oral cancer cells. Foods. 11:729. DOI: 10.3390/foods11050729. PMID: 35267362. PMCID: PMC8909074. PMID: 4f79d9c498b940108f6ce0191f054205. PMID: https://www.scopus.com/inward/record.uri?partnerID=HzOxMe3b&scp=85125954513&origin=inward.
26. Liu H, Wang GC, Zhang MX, Ling B. 2015; The cytotoxicology of momordicins I and II on Spodoptera litura cultured cell line SL-1. Pestic Biochem Physiol. 122:110–118. DOI: 10.1016/j.pestbp.2014.12.007. PMID: 26071815. PMID: https://www.scopus.com/inward/record.uri?partnerID=HzOxMe3b&scp=84930687173&origin=inward.
27. Nguyen TD, Schulze PC. 2020; Lipid in the midst of metabolic remodeling - therapeutic implications for the failing heart. Adv Drug Deliv Rev. 159:120–132. DOI: 10.1016/j.addr.2020.08.004. PMID: 32791076. PMID: https://www.scopus.com/inward/record.uri?partnerID=HzOxMe3b&scp=85090059792&origin=inward.
28. Bargui R, Solgadi A, Prost B, Chester M, Ferreiro A, Piquereau J, Moulin M. 2021; Phospholipids: identification and implication in muscle pathophysiology. Int J Mol Sci. 22:8176. DOI: 10.3390/ijms22158176. PMID: 34360941. PMCID: PMC8347011. PMID: bfbdd8ca3b224cb99bdde3c159b8b603. PMID: https://www.scopus.com/inward/record.uri?partnerID=HzOxMe3b&scp=85111369114&origin=inward.
29. Cai F, Ren F, Zhang Y, Ding X, Fu G, Ren D, Yang L, Chen N, Shang Y, Hu Y, Yi L, Zhang H. 2021; Screening of lipid metabolism biomarkers in patients with coronary heart disease via ultra-performance liquid chromatography-high resolution mass spectrometry. J Chromatogr B Analyt Technol Biomed Life Sci. 1169:122603. DOI: 10.1016/j.jchromb.2021.122603. PMID: 33690078. PMID: https://www.scopus.com/inward/record.uri?partnerID=HzOxMe3b&scp=85102054080&origin=inward.
30. Floegel A, Kühn T, Sookthai D, Johnson T, Prehn C, Rolle-Kampczyk U, Otto W, Weikert C, Illig T, von Bergen M, Adamski J, Boeing H, Kaaks R, Pischon T. 2018; Serum metabolites and risk of myocardial infarction and ischemic stroke: a targeted metabolomic approach in two German prospective cohorts. Eur J Epidemiol. 33:55–66. DOI: 10.1007/s10654-017-0333-0. PMID: 29181692. PMCID: PMC5803284. PMID: https://www.scopus.com/inward/record.uri?partnerID=HzOxMe3b&scp=85035082764&origin=inward.
31. Paradies G, Paradies V, Ruggiero FM, Petrosillo G. 2018; Mitochondrial bioenergetics and cardiolipin alterations in myocardial ischemia-reperfusion injury: implications for pharmacological cardioprotection. Am J Physiol Heart Circ Physiol. 315:H1341–H1352. DOI: 10.1152/ajpheart.00028.2018. PMID: 30095969. PMID: https://www.scopus.com/inward/record.uri?partnerID=HzOxMe3b&scp=85056619296&origin=inward.
32. Stamenkovic A, O'Hara KA, Nelson DC, Maddaford TG, Edel AL, Maddaford G, Dibrov E, Aghanoori M, Kirshenbaum LA, Fernyhough P, Aliani M, Pierce GN, Ravandi A. 2021; Oxidized phosphatidylcholines trigger ferroptosis in cardiomyocytes during ischemia-reperfusion injury. Am J Physiol Heart Circ Physiol. 320:H1170–H1184. DOI: 10.1152/ajpheart.00237.2020. PMID: 33513080. PMID: https://www.scopus.com/inward/record.uri?partnerID=HzOxMe3b&scp=85102721772&origin=inward.
33. Wang F, Liu S, Pei J, Cai L, Liu N, Liang T, Dong X, Cong X, Chun J, Chen J, Hu S, Chen X. 2020; LPA3-mediated lysophosphatidic acid signaling promotes postnatal heart regeneration in mice. Theranostics. 10:10892–10907. DOI: 10.7150/thno.47913. PMID: 33042260. PMCID: PMC7532668. PMID: https://www.scopus.com/inward/record.uri?partnerID=HzOxMe3b&scp=85092752613&origin=inward.
34. Wang Z, Greenbaum J, Qiu C, Li K, Wang Q, Tang SY, Deng HW. 2020; Identification of pleiotropic genes between risk factors of stroke by multivariate metaCCA analysis. Mol Genet Genomics. 295:1173–1185. DOI: 10.1007/s00438-020-01692-8. PMID: 32474671. PMCID: PMC7394724. PMID: https://www.scopus.com/inward/record.uri?partnerID=HzOxMe3b&scp=85085877083&origin=inward.
35. Moon SH, Liu X, Cedars AM, Yang K, Kiebish MA, Joseph SM, Kelley J, Jenkins CM, Gross RW. 2018; Heart failure-induced activation of phospholipase iPLA2γ generates hydroxyeicosatetraenoic acids opening the mitochondrial permeability transition pore. J Biol Chem. 293:115–129. DOI: 10.1074/jbc.RA117.000405. PMID: 29158256. PMCID: PMC5766913. PMID: https://www.scopus.com/inward/record.uri?partnerID=HzOxMe3b&scp=85040098448&origin=inward.
36. Shirai Y, Saito N. 2014; Diacylglycerol kinase as a possible therapeutic target for neuronal diseases. J Biomed Sci. 21:28. DOI: 10.1186/1423-0127-21-28. PMID: 24708409. PMCID: PMC4005014. PMID: https://www.scopus.com/inward/record.uri?partnerID=HzOxMe3b&scp=84899994000&origin=inward.
37. Mahajan S, Mellins ED, Faccio R. 2020; Diacylglycerol kinase ζ regulates macrophage responses in juvenile arthritis and cytokine storm syndrome mouse models. J Immunol. 204:137–146. DOI: 10.4049/jimmunol.1900721. PMID: 31801815. PMCID: PMC6920556. PMID: https://www.scopus.com/inward/record.uri?partnerID=HzOxMe3b&scp=85076876680&origin=inward.
38. Chambers KT, Cooper MA, Swearingen AR, Brookheart RT, Schweitzer GG, Weinheimer CJ, Kovacs A, Koves TR, Muoio DM, McCommis KS, Finck BN. 2021; Myocardial Lipin 1 knockout in mice approximates cardiac effects of human LPIN1 mutations. JCI Insight. 6:e134340. DOI: 10.1172/jci.insight.134340. PMID: 33986192. PMCID: PMC8262319. PMID: https://www.scopus.com/inward/record.uri?partnerID=HzOxMe3b&scp=85105966299&origin=inward.
39. Fujiwara A, Ozawa M, Sumida K, Hirawa N, Yatsu K, Ichihara N, Haze T, Komiya S, Ohki Y, Kobayashi Y, Wakui H, Tamura K. 2022; LPIN1 is a new target gene for essential hypertension. J Hypertens. 40:536–543. DOI: 10.1097/HJH.0000000000003046. PMID: 34772856. PMID: https://www.scopus.com/inward/record.uri?partnerID=HzOxMe3b&scp=85123969295&origin=inward.
40. Smeir E, Leberer S, Blumrich A, Vogler G, Vasiliades A, Dresen S, Jaeger C, Gloaguen Y, Klose C, Beule D, Schulze PC, Bodmer R, Foryst-Ludwig A, Kintscher U. 2021; Depletion of cardiac cardiolipin synthase alters systolic and diastolic function. iScience. 24:103314. DOI: 10.1016/j.isci.2021.103314. PMID: 34805785. PMCID: PMC8581512. PMID: https://www.scopus.com/inward/record.uri?partnerID=HzOxMe3b&scp=85122637574&origin=inward.
Fig. 1
Effect of momordicine I on cardiomyocyte viability.
(A) The chemical structural of momordicine I. (B) MTT assay showed the cardiomyocyte viability after treatment with different concentrations of momordicine I (i.e., 1.625, 3.125, 6.25, 12.5, 25.0 µg/ml) for 24 h. Data are shown as means ± SEM (n = 5). *p < 0.05 vs. control group.
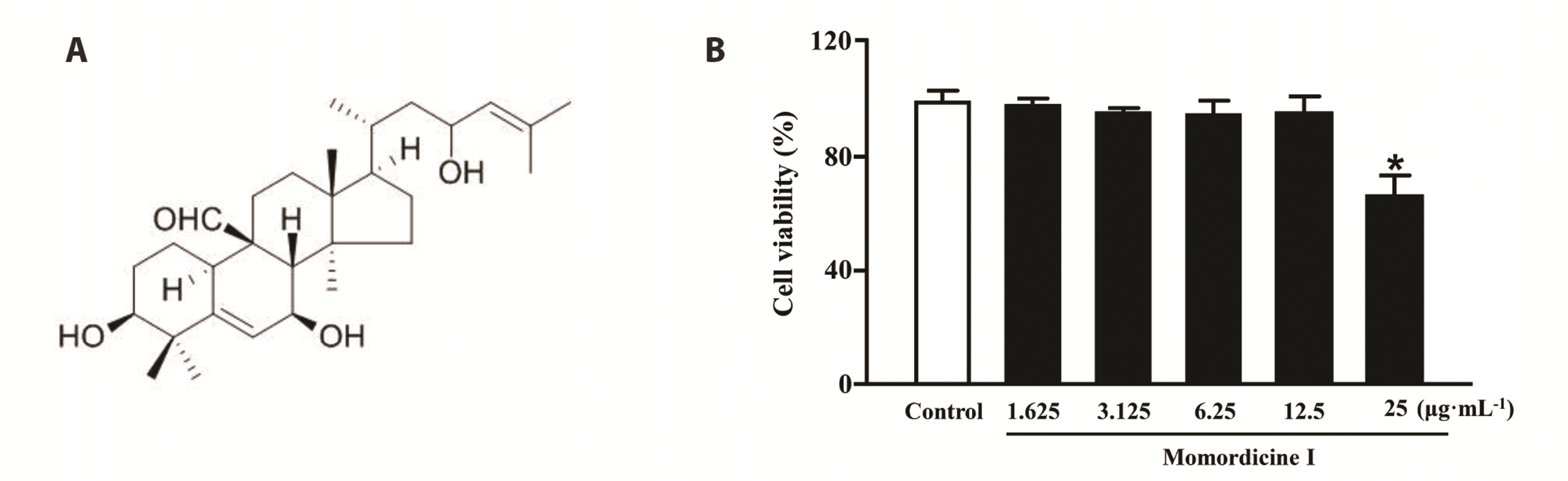
Fig. 2
Momordicine I inhibited isoproterenol-induced cardiomyocyte hypertrophy.
Cardiomyocytes were pretreated with momordicine I (12.5 µg/ml) for 1 h, and then exposed to isoproterenol (10 µM) for 24 h. (A) Representative microscopy images of each group were showed (100×). Momordicine I attenuated isoproterenol-induced marked increase in cell surface area (B), protein content (µg per 105 cells) (C), as well as expression of the hypertrophy-related fetal genes including ANP (D), β-MHC (E), and α-SKA (F). Data are shown as means ± SEM (n = 5). ISO, isoproterenol; I, momordicine I; ANP, atrial natriuretic peptide; β-MHC, β-myosin heavy chain; α-SKA, α-skeletal actin. *p < 0.05 vs. control group; #p < 0.05 vs. ISO group.
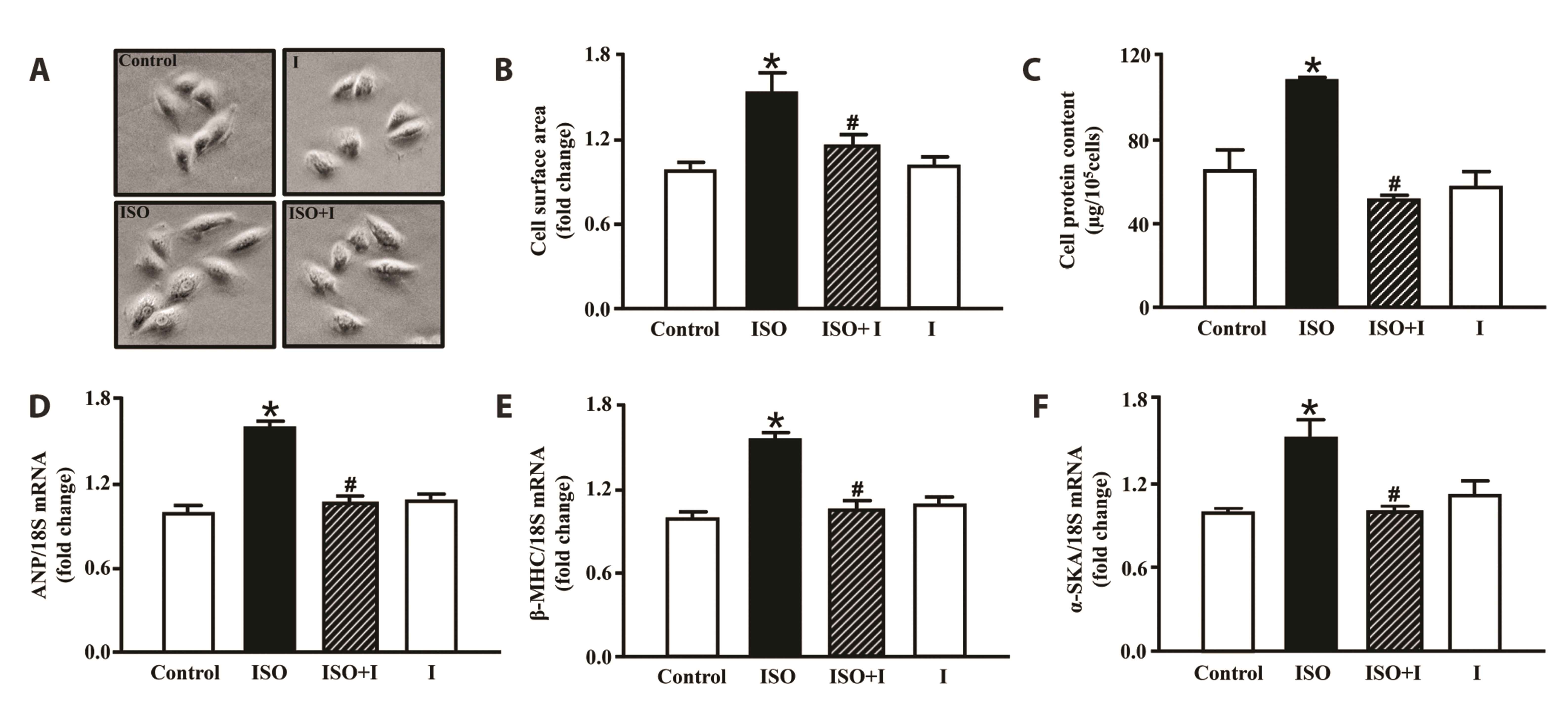
Fig. 3
Transcriptome sequencing analysis.
(A) Comparison of the number of upregulated and downregulated genes in three groups: Con vs. ISO, ISO vs. ISO + I, and Con vs. I. (B–D) Volcano plot of DEGs in three groups: Con vs. ISO, ISO vs. ISO + I, and Con vs. I. Con, control; ISO, isoproterenol; I, momordicine I; DEGs, differentially expressed genes.
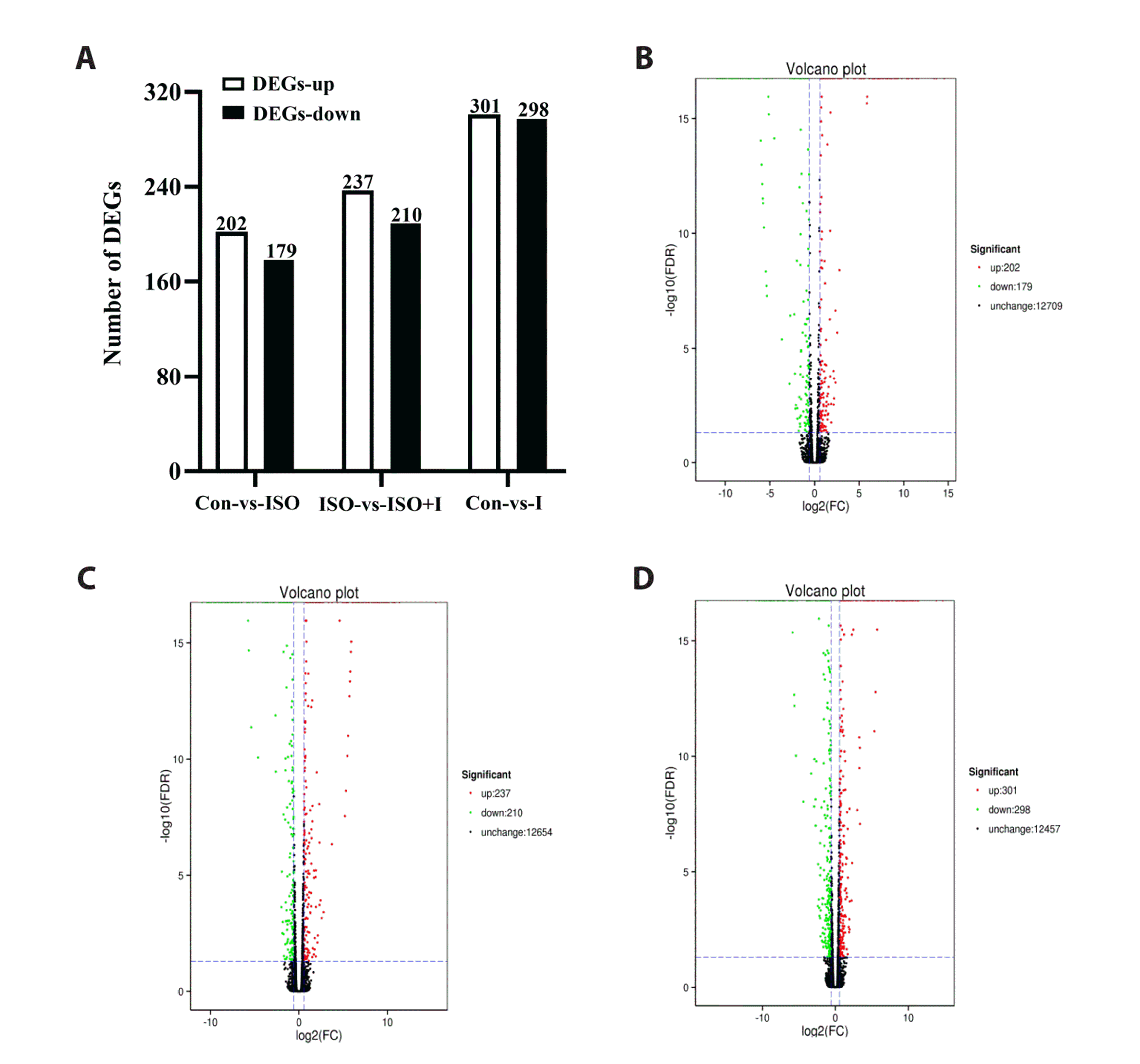
Fig. 4
Momordicine I inhibited isoproterenol-induced upregulation of PLA2G6 and DGK-ζ.
Cardiomyocytes were pretreated with momordicine I (12.5 µg/ml) for 1 h, and then exposed to isoproterenol (10 µM) for 24 h. RT-PCR showed that momordicine I prevented isoproterenol-induced increase in mRNA levels of PLA2G6 (A) and DGK-ζ (B) (n = 5). (C–E) Representative Western blot bands and quantitative analysis showed that momordicine I blunted isoproterenol-induced upregulation in protein expressions of PLA2G6 and DGK-ζ (n = 6). Data are shown as means ± SEM. ISO, isoproterenol; I, momordicine I; PLA2G6, group VI phospholipase A2; DGK-ζ, diacylglycerol kinase ζ. *p < 0.05 vs. control group; #p < 0.05 vs. ISO group.
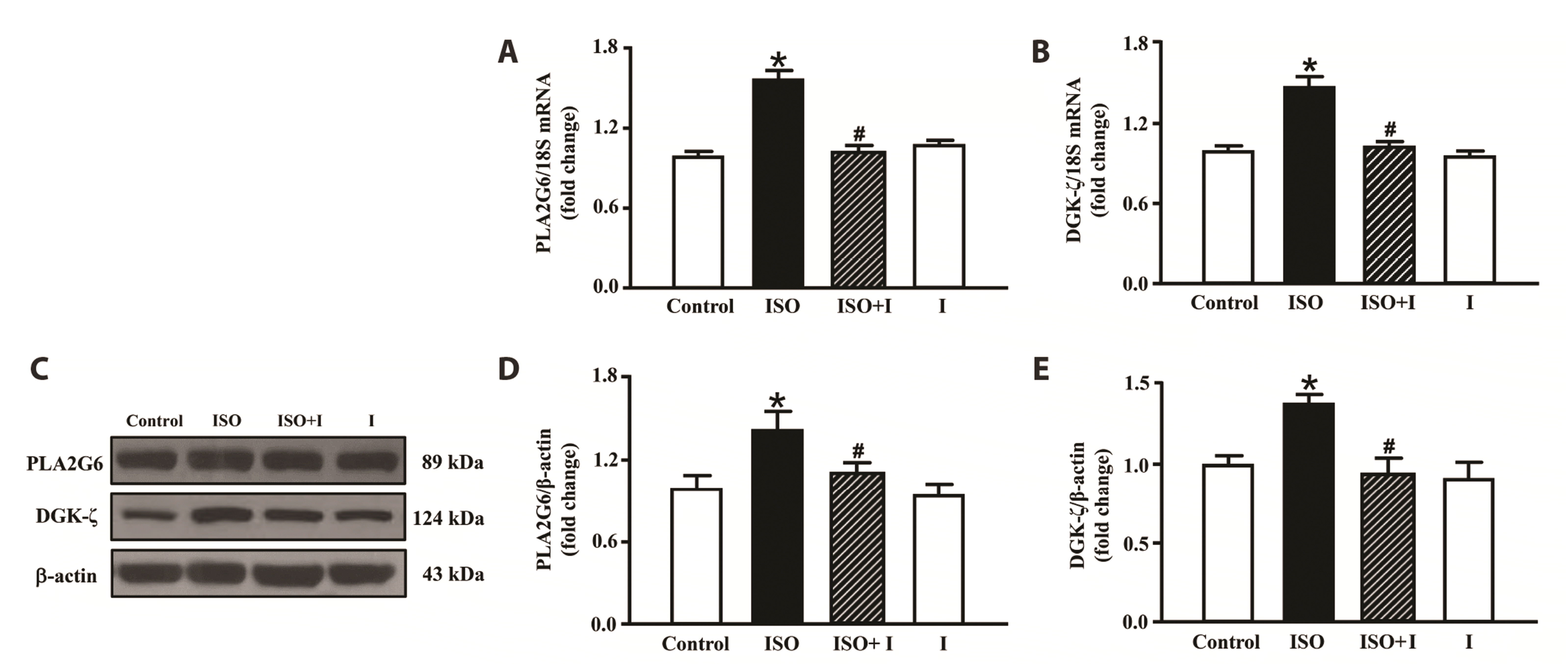
Table 1
Primer sequences and lengths for fluorescence quantitative PCR
Table 2
Statistical results of the top 10 items in each functional category of differential expression genes