Abstract
It has been reported that stressful events in early life influence behavior in adulthood and are associated with different psychiatric disorders, such as major depression, post-traumatic stress disorder, bipolar disorder, and anxiety disorder. Maternal separation (MS) is a representative animal model for reproducing childhood stress. It is used as an animal model for depression, and has well-known effects, such as increasing anxiety behavior and causing abnormalities in the hypothalamic-pituitary-adrenal (HPA) axis. This study investigated the effect of MS on anxiety or aggression-like behavior and the number of GABAergic neurons in the hippocampus. Mice were separated from their dams for four hours per day for 19 d from postnatal day two. Elevated plus maze (EPM) test, resident-intruder (RI) test, and counted glutamic acid decarboxylase 67 (GAD67) or parvalbumin (PV) positive cells in the hippocampus were executed using immunohistochemistry. The maternal segregation group exhibited increased anxiety and aggression in the EPM test and the RI test. GAD67-positive neurons were increased in the hippocampal regions we observed: dentate gyrus (DG), CA3, CA1, subiculum, presubiculum, and parasubiculum. PV-positive neurons were increased in the DG, CA3, presubiculum, and parasubiculum. Consistent with behavioral changes, corticosterone was increased in the MS group, suggesting that the behavioral changes induced by MS were expressed through the effect on the HPA axis. Altogether, MS alters anxiety and aggression levels, possibly through alteration of cytoarchitecture and output of the ventral hippocampus that induces the dysfunction of the HPA axis.
Many lines of evidence, including epidemiologic studies from human beings and extensive animal experimental data, indicate that early life events play an essential role in influencing later behavioral and emotional responses to stressors [1-4]. Although the mechanism of how early life stress changes the behavioral and/or emotional outcome remains unclear, a recent explanation for this is that adverse early life experiences can change neural connectivity in the underlying brain network [5-9]. For example, abnormal maternal care and chronic early life stress change the number and function of neuronal synaptic activities in different brain areas, including the hypothalamus, hippocampus, septal area, etc. [10-13]. These lines of evidence suggest that maternal separation (MS) in early life may hinder the normal neural circuitry of the brain, resulting in emotional disorders such as anxiety and aggressive behavior.
The hippocampus, belonging to the limbic system, is a primitive cortical structure that plays an essential role in memory processing and spatial cognition, including how mammals learn to understand and navigate the environment. For this reason, several researchers have long focused on its role in spatial learning memory and dementia, especially concerning Alzheimer’s disease [14-18]. However, literature has also shown that the hippocampus takes part in memory and intimately in emotion to regulate a range of stress responses [19-21]. In humans, the decline in the volume of the hippocampus and its resulting dysfunction are related to psychological disorders, such as post-traumatic stress disorder, bipolar disorder, and depression [22-26]. These two distinct functional roles of the hippocampus can be explained by dividing it into two separate regions, namely, the dorsal hippocampus and the ventral hippocampus [10,27-29]. The dorsal hippocampus receives polymodal sensory inputs from the cortical areas. It is involved mainly in learning and memory, while the ventral hippocampus is more closely linked to subcortical limbic areas (hypothalamus, amygdala, etc.) and is mainly associated with the modulation of reward circuitry and emotional behavior [10,30-32].
Many lines of evidence have shown that hippocampal GABAergic interneurons are associated with mood disorders’ development [33-35]. The interneurons control the activity levels of principal neurons by gating the information flow at the synaptic cleft [10,36-38]. Although interneurons possess the largest diversity in morphology and physiological properties, Most interneurons in the central nervous system are inhibitory interneurons, which characteristically release gamma-aminobutyric acid as their neurotransmitter [39]. The hippocampus is a very susceptible structure to the effects of different stressors and is known to be involved in fear, anxiety, or aggression through direct projection reaching multiple areas of the brain, such as the hypothalamus, prefrontal cortex, basal amygdala, and nucleus accumbens [40-44]. Particularly, the hippocampus sends projections to the hypothalamic paraventricular nucleus (PVN) for involvement in the regulation of the hypothalamic-pituitary-adrenal (HPA) axis [35,45,46].
It was previously shown that early life stress, especially MS, can decrease long-term potentiation and release probability of Mossy fiber-CA3 synapses at the ventral hippocampus in neonatal mice, which may result in behavioral disorders in the adolescent period [47]. These electrophysiological and behavioral data hint at a correlation between developmental changes in the ventral hippocampus caused by early life stress and psychiatric illness. In this study, we aimed to determine whether adolescent behavioral disorders following early MS were caused by alterations in the cytoarchitecture of the ventral hippocampus.
The adult male C57BL/6 and Balb/c mice (Samtako Inc., Osan, Korea) were used. The C57BL/6 mice were used for all experimental procedures, and the Balb/c mice were used only as an intruder in the RI test. All animals were housed in a standard cage with sterilized food and tap water available ad libitum and maintained on a 12-h:12-h light-dark cycle (light on from 7:00 AM) under constant room temperature (21°C ± 2°C). All experimental procedures were conducted in accordance with the National Institutes of Health Guide for the Care and Use of Laboratory Animals (NIH Publication No. 80-23, revised 1996) with the approval of the Institutional Animal Research Ethics Committee of Eulji University (approval No. EUIACUC14-13).
The MS experiments were performed using the same procedures as in our previous study [47,48]. Briefly, the male and female mice were mated to produce litters of 8–12 pups. After birth, the pups were randomly divided into two groups: the MS group and the handling (HD) group. The pups in the MS group were separated from their dams for four hours (10:00–14:00) per day from the postnatal day (PND) 2–20. During the separation, the pups were placed in another isolated room with heated, clean sawdust (29°C ± 1°C) to avoid communicating with their dams through ultrasound vocalization and their dams. The pups in the HD group remained in their home cage with their dams and littermates during the four-hour separation, except briefly moving them to a clean cage and returning to their home cage. This HD procedure, never lasting for more than five minutes per litter, simulates the handling undergone by MS pups and serves as a control [49,50]. Only one proficient researcher conducted all the MS procedures to prevent potential experimental bias. Other researchers were blocked from accessing the cages.
After weaning at PND 21, all pups were regrouped by gender at PND 22, and all subsequent procedures were conducted using males only. The behavioral changes of MS during the adolescence period were evaluated by accessing the elevated plus maze (EPM) test and RI test. EPM test was conducted between PND 42 and PND 46. RI test was conducted at PND 49 following single-housing of animals for two weeks from PND 35. Corticosterone (CORT) assay and immunohistochemistry (IHC) for GAD67 and parvalbumin (PV) experiments were conducted between PND 42 and 49 (Fig. 1). A total number of 68 MS and 68 HD was used in this study: EPM test (10 MS and 10 HD), RI test (10 MS and 10 HD), CORT assay (10 MS and 10 HD), IHC (13 MS and 13 HD).
The EPM apparatus is a plus-shaped acryl maze with two opposite open arms and two opposite closed arms, extending out from a central platform. The open arm is 65 × 5-cm, the closed arm is 65 × 5-cm, and the height is 15-cm. Animals were placed in the center platform of the maze facing one of the open arms and then allowed to explore the open or closed arms for ten minutes. The time spent in the different arms was recorded with the Ethovision Video Tracking System (Noldus Inc., Wageningen, Netherlands).
For the RI test, from PND 35, each MS and HD mouse was single-housed in an individual home cage for 14 days. The bedding was not changed for four days (from PND 45 to PND 49) before testing. On the test day (at PND 49), the other mouse species (Balb/c) of the same age were placed into the home cage as an intruder. For ten minutes, aggressive behavior of the host mice were recorded using the Ethovision Video Tracking System. The latency, total duration, and the number of attacks on the host mouse were observed and assessed.
Anesthesia was induced using isoflurane (Isoflurane; Hana Pharm Co., Hwaseong, Korea) in an empty induction chamber. Following rapid decapitation, trunk blood was immediately obtained from animals, and the obtained blood (about 0.7-ml) was allowed to clot at room temperature for 30 min. After centrifugation (2,300 rpm, five min), plasma was transferred into fresh tubes and stored at –80°C until used for CORT analysis. Blood sampling occurred between 09:00 AM and 11:00 AM.
The serum levels of CORT were measured using Cayman’s Corticosterone EIA Kit according to the manufacturer’s instructions (Cayman chemical, Ann Arbor, MI, USA) as described previously by research groups [51-55]. Briefly, 100-μl standards or samples were added in duplicate to the wells of the microtiter plate. Assay buffer (200-μl) was added to the nonspecific binding (NSB) wells, and 100-μl assay buffer was added to wells to act as maximum binding wells. Then, 50-μl conjugate and 50 μl of CORT antibody (except the NSB wells) were added to each well and the plate was incubated for two hours at room temperature on a horizontal orbital microplate shaker (450–550-rpm). After the plate was washed thrice using the Washing Solution and the remaining Washing Solutions were completely removed by decantation and aspiration. Then, 200-μl p-nitro phenyl phosphate substrate was added to each well and incubated for one hour at room temperature without shaking. The optical density of the enzyme products was read at 405-nm using a plate reader (SPECTRO star Nano; BMG Labtech, Ortenberg, Germany) within 15 min after the reaction was terminated by adding 50-μl Stop Solution. The values of samples were calculated according to the standard curve (BMG Labtech).
Animals were anesthetized through an intraperitoneal injection mixture of ketamine (70-mg/kg) and rompun (8-mg/kg) and transcardially perfused with physiological saline, followed by 150-ml 4% paraformaldehyde in phosphate-buffered saline (PBS). Brains were eliminated immediately, post-fixed in the same fixative for two hours, and infiltrated with 30% sucrose solution overnight at 4°C until they sank. The whole brains were rapidly frozen in 2-methylbutane chilled on dry ice and mounted in Tissue-Tek OCT compound (Sakura Finetechnical Co., Tokyo, Japan). Serial horizontal sections of 40-μm-thicknesses were obtained on a Cryostat Microtome (Leica Microsystems Inc., Wetzlar, Germany). Every sixth section, at a periodicity of 240-μm, was obtained as one set, so six sets were prepared per brain. Before immunohistochemical staining, the sections of a set were scanned under a stereomicroscope (Nikon Inc., Tokyo, Japan) to select specimens, including a section passing through the ventral hippocampus between level Interaural 3.12–2.04-mm according to Paxinos and Watson [56]. A total of six specimens per brain were selected, and immunostaining was conducted.
IHC staining was executed using the free-floating method. The horizontal sections were washed for ten minutes in 0.1 M PBS, and endogenous peroxidase activity was quenched by incubating the tissue sections with 0.3% hydrogen peroxide in PBS for 30 min. After washing, the sections were incubated in PBS containing 0.1% Triton X-100 (PBST) and 10% normal horse serum for one hour to reduce nonspecific staining. The sections were then incubated with primary antibodies at 4°C overnight. The primary antibodies used in this study were mouse anti-GAD67 monoclonal antibody (1:1,000; Chemicon, Temecula, CA, USA) and mouse anti-PV monoclonal antibody (1:1,000; Sigma-Aldrich, St. Louis, MO, USA). The sections were washed thrice with PBST and incubated for two hours with biotinylated horse anti-mouse immunoglobulin G (1:200; Vector Laboratories Inc., Burlingame, CA, USA), followed by incubation for one hour with Avidin-Biotin Peroxidase Complex (Vector Laboratories Inc.). Antigens were visualized with 3, 3’-diaminobenzidine tetrahydrochloride (Sigma-Aldrich) solution containing 0.01% hydrogen peroxide. Sections were mounted on Vectabond (Vector Laboratories Inc.) coated slides, counterstained with hematoxylin, dehydrated through a graded ethanol series, cleared in xylene, and covered with coverslips using paramount (Fisher Scientific, Pittsburgh, PA, USA).
The number of GAD67- or PV-immunoreactive (-ir) neurons in the ventral hippocampus was counted using a researcher blind to the experimental conditions. Light photomicrography images were collected on an Olympus Ax 70 microscope (Olympus Inc., Tokyo, Japan) equipped with a Carl Zeiss AxioCam MRC digital camera (Carl Zeiss Inc., Jena, Germany) and AxioVision image capture software (version 4.6; Carl Zeiss Inc.). The researcher traced contours within the boundaries of each ventral hippocampal subfield (dentate gyrus [DG], CA3, and CA1) at 4× magnification. The border between each region was defined by Cappaert’s method [57]. Its coiled structure can distinguish the DG with an opened concave part directed towards the hippocampus proprius. The hippocampus proprius is subdivided into two subfields, CA1 and CA3, according to the density, size, and branching of axons and dendrites of pyramidal cells. CA3 is the region with large, less densely packed cells, and CA1 is the region with densely packed medium-sized cells. In this study, we did not include the field CA2, a narrow transitional field between CA3 and CA1.
Cells of GAD67-ir or PV-ir were then counted at 10× magnification using a meander scan profile counting technique. The meander scan profile counting method is a semi-automated scanning method that allows the user to view and count all cells within the traced contour. For a more precise comparison, we further divided each hippocampal subfield into several layers [57]. The DG was further divided into three layers: granular cell layer (gcl), hilus, and molecular cell layer (mcl). CA3 was further divided into five layers: stratum oriens (so), stratum pyramidale (sp), stratum lucidum (sl), stratum radiatum (sr), and stratum moleculare (slm). The CA1 was also further divided into four layers: so, sp, sr, and slm. To determine the density of immunoreactive cells in a given region of interest, we divided the total number of immunoreactive cells in that region by the total size of the traced contour (in mm2). Our cell counts are expressed as the average number of cells per mm2.
To examine the effect of neonatal MS on behavioral characteristics in the adolescent period, two groups were assessed using the EPM test and the RI test. In the EPM test, the MS mice spent significantly less time in the open arms (HD: 65.28 ± 11.06 sec; MS: 35.29 ± 7.49 sec, p = 0.035; Fig. 2A) and spent more time in the closed arms (HD: 466.95 ± 14.02 sec; MS: 515.85 ± 15.75 sec, p = 0.030; Fig. 2B) compared to the HD group. Simultaneously, the ratio of entries into the closed arm section in the MS group was more than that of the HD mice (HD: 68.04 ± 1.73; MS: 78.24 ± 3.956, p = 0.034; Fig. 2D). These behavioral features show more anxiety-like properties in the MS mice. No significant differences were observed in the duration time in the center between the two groups (HD: 40.7 ± 3.23 sec; MS: 33.11 ± 6.13 sec, p = 0.297. data now shown). Moreover, no differences were shown in the total number of entries into all arms (HD: 27.3 ± 2.14 sec; MS: 20.77 ± 2.68 sec, p = 0.071. data now shown). These results indicate that the behavioral effects are probably unrelated to changes in activity levels.
In the RI test, the MS mice exhibited a short latency to attack intruder mice (latency: HD: 448.20 ± 96.90 sec; MS: 323.20 ± 65.62 sec, p = 0.028; Fig. 2E). Meanwhile, the MS mice exhibited significant elevated time in the total duration of attacks (HD: 8.12 ± 10.22 sec; MS: 42.23 ± 15.32 sec, p = 0.007; Fig. 2F) and increased the number of attacks (HD: 4 ± 2.84 sec; MS: 23.67 ± 5.38 sec, p = 0.001; Fig. 2G) compared with the HD mice. These results show that MS mice have more aggressive behavior than HD mice.
Based on the observed behavioral phenotypes in the MS group, basal CORT was examined to determine whether neonatal MS leads to an alternation of it. Fig. 2H showed elevated baseline CORT levels in MS mice compared to HD mice (HD: 66.14 ± 6.77-mg/ml; MS: 88.00 ± 8.89 mg/ml, p = 0.031).
The numerical densities of GAD67-ir neurons in vHipp were estimated, and their differences between MS and HD mice were statistically assessed (Figs. 3 and 4). The total number of GAD67-ir neurons per unit area (mm2) in DG, CA3, and CA1 (Fig. 3), subiculum, presubiculum, parasubiculum (Fig. 4) was significantly higher in MS mice than in HD mice (DG: HD: 222.92 ± 6.54; MS: 333.17 ± 10.46, p < 0.001; CA3: HD: 268.95 ± 3.24; MS: 387.62 ± 3.60, p < 0.001; CA1: HD: 189.53 ± 3.29; MS: 261.86 ± 6.61, p < 0.001; subiculum: HD: 156.39 ± 5.15; MS: 211.45 ± 6.91, p < 0.001; presubiculum: HD: 348.78 ± 9.185; MS: 589.95 ± 13.048, p < 0.001; parasubiculum: HD: 124.22 ± 6.57; MS: 184.50 ± 8.07, p < 0.001). The values for GAD67-ir neurons of each sublayer are presented in Figs. 3 and 4.
PV IHC staining of the ventral hippocampus indicated that the number of immune-reactivity cells per hippocampal area was significantly higher in the MS than the HD designed (Figs. 5 and 6). Similar results to GAD67, in the most regions the total number of PV-ir neurons in the MS group showed significantly higher than the HD group (DG: HD: 222.92 ± 1.12; MS: 333.17 ± 2.37, p < 0.001; CA3: HD: 90.48 ± 1.74; MS: 160.76 ± 3.59, p < 0.001; CA1: HD: 87.15 ± 3.09; MS: 109.91 ± 3.93, p < 0.001; presubiculum: HD: 282.43 ± 13.75; MS: 452.79 ± 18.97, p < 0.001; parasubiculum: HD: 42.45 ± 3.99; MS: 132.37 ± 13.22, p < 0.001). However, in the subiculum region (Fig. 6), there were no significant differences between the two groups (CA1: subiculum: HD: 72.27 ± 6.03; MS: 86.29 ± 6.87, p = 0.133).
In this study, the mice with neonatal MS exhibited increased anxiety-like/aggressive behavior in adolescence. This behavioral change was accompanied by an increase in the CORT level, suggesting that MS-induced anxiety and aggressive behavior may be because of changes in the HPA axis. Additionally, GAD67-positive cells increased in most hippocampus regions, such as DG, CA3, CA1, and subiculum, and parvalbumin-positive cells increased in DG and CA3.
There are five types of rodent models have been used to investigate the mechanisms linking early life stress to behavioral outcomes (mood disorders) in adulthood; 1) prenatal stress exposure, 2) acute maternal deprivation procedures, 3) chronic or periodic MS model, 4) chronic early life stress, 5) early weaning of the pups [58]. Among them, the most widely used manipulation to manufacture a rodent model of early life stress is MS. When tested in adults, rodents subjected to MS show enhanced anxiety and/or depressive behavior, deficits in learning and memory, enhanced expression of corticotropin-releasing factor, and alterations of mossy fiber density in the CA3 region of the hippocampus [59]. Because the adolescent period is a vital period for physical and mental development, the behavioral, hormonal, and neuro-architectural changes caused by MS could be facilitated and entrenched in this period. Although this is important, only a few studies have directly examined MS effect on adolescents [60]. This study aimed to examine the impact of MS on the behavioral, hormonal, and neuro-architectural changes in adolescent C57BL/6 mice.
Since rodents have innate fear of open spaces [61], the EPM is considered a gold-standard behavioral assay for anxiety-like behavior. Meanwhile, the RI test is widely used to evaluate aggressive tendencies because many species display territorial aggression against intruders in defense of mates and/or offspring [62]. In the EPM test and RI test, the MS exhibited increased anxiety and aggression in the adolescent period. These behavioral features of MS agree with other studies, showing MS-induced increased emotionality in male rodents [47]. There are a considerable amount of experimental evidence, which indicates the gender differences in the anxiety-like behavior and stress responses [63]. Usually, male rodents are more vulnerable to early life stress, specifically in anxiety-like behavior, than females. For example, Ito et al. [64] showed the sexually dimorphic patterns in stress responses by showing higher autonomic and anxiety-like behavior in male than in female rodents. They exhibited a lower frequency of entry into and shorter stay in the open arms in the EPM test and an exacerbation of autonomic responses, such as stress-induced hyperthermia and tachycardia, in the early-weaned male rats compared with the normally weaned or early-weaned female rats. For these reasons, this study was conducted using only male mice to avoid confounding issues due to sex.
Although the MS has also been proposed as a depression animal model [65-67], phenotypes other than anxiety, such as depression, did not appear in our MS study (data not shown). It is unclear why MS causes different behavior phenotypes (anxiety/aggression vs. depression) based on the researchers. This difference may be because of a different species background, the duration of separation time, feeding environment and housing conditions, and so on [68]. Therefore, efforts were made to rule out possible factors, such as temperature, ultrasound vocalization, and odors, by keeping many qualifications. We used warm bedding and an isolated room when pups were separated from their dams, and only one proficient researcher was allowed access to the area. As a result, our MS model has consistently shown stable and reliable behavioral results, so this work focuses on increasing anxiety and aggression using MS during the adolescent period. Following these behavioral results, the CORT serum level in adolescent MS mice was compared with the MD.
Excitation-inhibition imbalance (E-I imbalance) is frequently associated with a range of neurological diseases and disorders, such as epilepsy [69], schizophrenia [70], Alzheimer’s disease [71], and autism spectrum disorder [72]. The long-lasting changes in brain interneuron populations have been proposed to contribute to the pathophysiology of many important neuro-psychiatric disorders [73] since it causes E-I imbalance. Furthermore, inhibitory interneurons have been thought to affect the net output of the projection and influence local neural circuits, and contribute to precise regulation of the timing of action potentials. It has been reported that changes in the ventral hippocampal activity owing to changes in E-I balance are related to physiological or pathological anxiety and fear [74-76]. Particularly, the projection from the ventral CA1 and subiculum to the PVN has an anxiolytic effect by inhibiting the HPA axis [77,78], and it has been reported that projection to the lateral septum also decreases anxiety when activated [79]. Alternatively, the excitation of projection neurons to the lateral hypothalamus [80] or DG granule cells [81] increases anxiety, and the hippocampus plays a vital role in the overall anxiety level. The role varies based on the region and the projection pathway. Therefore, there was an attempt to investigate whether the behavioral change was caused by the alteration of the GABAergic population and whether the effect on the population differs for each hippocampus region.
Among different types of GABAergic interneurons, changes were examined in GAD67-expressing interneurons since different kinds of literature have noted GAD67 protein as the molecule most associated with different types of neuropsychiatric disorders [82]. To explore this issue, the number of GAD67 interneurons was investigated in other subfields of the ventral hippocampus of MS and HD. The IHC data showed that the populations of GAD67 interneurons in the ventral hippocampus in MS were 1.3-fold higher than in the HD (Figs. 3 and 4).
Among the diverse subtypes of GABAergic interneurons (PV, somatostatin, calbindin, and neuropeptide-Y) populating the hippocampal formation, PV-positive interneurons play a critical role in emotional states, stress response, and cognitive functions [83]. The PV interneurons are mostly fast-spiking inhibitory neurons that control the circuitry activity of pyramidal cells [10]. Many lines of evidence indicated that the number or density of PV interneurons in the hippocampus is significantly reduced in depression animal models [84,85]. This study showed that the number of PV interneurons in the ventral hippocampus, especially in the DG and CA3 regions, was significantly increased about 2.0-fold in MS compared with HD (Figs. 5 and 6). In the subiculum region, which greatly influences the HPA axis, the change in PV interneurons was insignificant and GAD67-expressing neurons increased. This suggests that the increase in CORT level and anxiety caused by MS is likely due to GABAergic neurons other than PV interneurons.
The hippocampal formation includes the hippocampal gyrus (CA1, CA3, etc.) and the DG. Different subfields of the hippocampus may perform different functions and may also be linked with various mental dysfunctions [30,86,87]. Furthermore, each subfield of the hippocampus has a distinct topographical layer organization and layer-specific connections [88]. In this study, there is an attempt to accurately estimate the number of GAD67 and PV interneurons in different subfields of the hippocampus and each layer. As a result, an increase in the GABAergic population was observed in regions known to reduce anxiety, such as the subiculum, and in regions, such as DG, which increase anxiety when excitability is increased.
Most reports on the effects of each hippocampus on anxiety have only investigated the contribution to the anxiety of a specific population through resection of that region or inhibition of a specific projection using optogenetics. In this study, overall changes in hippocampal GABAergic or PV-positive neuron populations caused by MS were observed. For more physiological conditioning events, such as MS, it is challenging to determine the extent of the effects of projection pathways or neuron subtypes on anxiety for now. The ventral subiculum sends projections to the paraventricular nucleus, a component of the HPA axis that produces corticotropin-releasing hormone, and suppresses the HPA axis activity directly [77] or indirectly [46,89]. The HPA axis is a crucial neuroendocrine stress response system linking the brain with cortisol secretion from the adrenal cortex [90,91]. If the output of the subiculum is inhibited, it can be expected that the HPA axis function will be enhanced and the anxiety and aggression levels will be altered upward [92]. Our data showed an increased number of GAD67 and PV interneurons in MS (Figs. 3–6). The disinhibition circuits to the HPA axis from the hippocampus and/or subiculum can be attributed to increased inhibition of GABAergic interneurons in MS, leading to the increased basal level of serum cortisol and anxiety- and aggressive-phenotype in adolescence. DG act as the main gateway for input in the hippocampus. Regarding anxiety and fear, DG distinguishes context and helps to selectively display fear and anxiety responses in appropriate contexts [81]. When the activity of DG is reduced, the ability to discriminate context is decreased, and fear and anxiety can be generalized [93,94], so it can be expected that an increase in anxiety response will be observed. However, this role of the DG in anxiety is less direct than the subiculum’s function of buffering anxiety responses through the HPA axis. Alternatively, when the physiological conditions of MS are applied, the reduction in output owing to the increase in the GABAergic population of the subiculum directly affects anxiety and aggression through the HPA axis, so it may overwhelm the reduction in output in other parts and have a decisive effect on the behavioral change. In future studies, it is expected that this hypothesis can be confirmed through selective inhibition experiments through techniques, such as optogenetics for the subiculum region with MS. Additionally, it is expected that the description of the GABAergic and PV populations by region and layer will be used in subsequent studies.
Compared with anxiety, little has been reported so far on the effect of the hippocampus on aggression. An association between hippocampal atrophy and increased aggressive behavior has been reported in patients with borderline personality disorder [95]; recently, it has been reported that the ventral hippocampus increases aggression via the ventromedial hypothalamus (VMH) [44]. However, our data indicate an association between an increase in inhibitory neurons and an increase in aggression in the ventral hippocampus, unlike previously reported. It is well known through several reports that the ventral hippocampus, especially the subiculum region, inhibits the activation of the PVN and HPA axes. Alternatively, the recent report that the ventral hippocampal activity increases aggression [44] suggests a role for excitatory projections from the ventral hippocampus to the VMH. This difference may be induced by the difference between the indiscriminate inhibition of the entire ventral hippocampal projection toward the VMH and physiological conditioning, such as MS. Alternatively, it can be explained that when MS is applied, as in anxiety, the inhibition of the subiculum-PVN pathway plays the most decisive role, leading to increased aggression. It is expected that this study will clarify the role of the hippocampus in physiological or pathological changes in aggression, as the effects of the output of numerous pathways starting from the hippocampus on aggression are demonstrated in subsequent studies.
As mentioned in INTRODUCTION, it was previously shown that decreased LTP at the ventral hippocampus CA3 in neonatal MS mice [47], and proposed the possibility that early life stress may influence the developmental neural network of the ventral hippocampus. This study revealed the effect of MS on neural circuits through changes in the number of GABAergic neurons in each region of the hippocampus. In the future, to investigate the functional effects of these changes, it is necessary to examine changes in excitatory and inhibitory signals and changes in synaptic plasticity through electrophysiological recordings in each hippocampal region, especially in the subiculum. Additionally, the increase in IPSC and subsequent decrease in LTP are usually accompanied by changes in the electrophysiological microenvironment in the brain region, particularly alterations in the kinetics of interneurons [96]. Therefore, there is a requirement to look at alterations in the kinetics of interneurons in the ventral hippocampus because the migration and distribution of the brain interneurons can be affected during brain development by early life stress [97].
Notes
REFERENCES
1. Cirulli F, Francia N, Berry A, Aloe L, Alleva E, Suomi SJ. 2009; Early life stress as a risk factor for mental health: role of neurotrophins from rodents to non-human primates. Neurosci Biobehav Rev. 33:573–585. DOI: 10.1016/j.neubiorev.2008.09.001. PMID: 18817811. PMCID: PMC2692357. PMID: https://www.scopus.com/inward/record.uri?partnerID=HzOxMe3b&scp=61849179516&origin=inward.
2. Kikusui T, Takeuchi Y, Mori Y. 2004; Early weaning induces anxiety and aggression in adult mice. Physiol Behav. 81:37–42. DOI: 10.1016/j.physbeh.2003.12.016. PMID: 15059682. PMID: https://www.scopus.com/inward/record.uri?partnerID=HzOxMe3b&scp=1842418490&origin=inward.
3. Stone LL, Otten R, Soenens B, Engels RC, Janssens JM. 2015; Relations between parental and child separation anxiety: the role of dependency-oriented psychological control. J Child Fam Stud. 24:3192–3199. DOI: 10.1007/s10826-015-0122-x. PMID: 26472930. PMCID: PMC4598341. PMID: https://www.scopus.com/inward/record.uri?partnerID=HzOxMe3b&scp=84943349722&origin=inward.
4. Veenema AH, Neumann ID. 2009; Maternal separation enhances offensive play-fighting, basal corticosterone and hypothalamic vasopressin mRNA expression in juvenile male rats. Psychoneuroendocrinology. 34:463–467. DOI: 10.1016/j.psyneuen.2008.10.017. PMID: 19056182. PMID: https://www.scopus.com/inward/record.uri?partnerID=HzOxMe3b&scp=59249096603&origin=inward.
5. Ellenbroek BA, van den Kroonenberg PT, Cools AR. 1998; The effects of an early stressful life event on sensorimotor gating in adult rats. Schizophr Res. 30:251–260. DOI: 10.1016/S0920-9964(97)00149-7. PMID: 9589519. PMID: https://www.scopus.com/inward/record.uri?partnerID=HzOxMe3b&scp=0032502849&origin=inward.
6. Walker EF, Diforio D. 1997; Schizophrenia: a neural diathesis-stress model. Psychol Rev. 104:667–685. DOI: 10.1037/0033-295X.104.4.667. PMID: 9337628. PMID: https://www.scopus.com/inward/record.uri?partnerID=HzOxMe3b&scp=0031255540&origin=inward.
7. Llorente R, O'Shea E, Gutierrez-Lopez MD, Llorente-Berzal A, Colado MI, Viveros MP. 2010; Sex-dependent maternal deprivation effects on brain monoamine content in adolescent rats. Neurosci Lett. 479:112–117. DOI: 10.1016/j.neulet.2010.05.039. PMID: 20493239. PMID: https://www.scopus.com/inward/record.uri?partnerID=HzOxMe3b&scp=77953913552&origin=inward.
8. Llorente R, Villa P, Marco EM, Viveros MP. 2012; Analyzing the effects of a single episode of neonatal maternal deprivation on metabolite profiles in rat brain: a proton nuclear magnetic resonance spectroscopy study. Neuroscience. 201:12–19. DOI: 10.1016/j.neuroscience.2011.11.033. PMID: 22120435. PMID: https://www.scopus.com/inward/record.uri?partnerID=HzOxMe3b&scp=84855520370&origin=inward.
9. Tóth M, Halász J, Mikics E, Barsy B, Haller J. 2008; Early social deprivation induces disturbed social communication and violent aggression in adulthood. Behav Neurosci. 122:849–854. DOI: 10.1037/0735-7044.122.4.849. PMID: 18729638. PMID: https://www.scopus.com/inward/record.uri?partnerID=HzOxMe3b&scp=50849107709&origin=inward.
10. Fanselow MS, Dong HW. 2010; Are the dorsal and ventral hippocampus functionally distinct structures? Neuron. 65:7–19. DOI: 10.1016/j.neuron.2009.11.031. PMID: 20152109. PMCID: PMC2822727. PMID: https://www.scopus.com/inward/record.uri?partnerID=HzOxMe3b&scp=73549123839&origin=inward.
11. Fabricius K, Wörtwein G, Pakkenberg B. 2008; The impact of maternal separation on adult mouse behaviour and on the total neuron number in the mouse hippocampus. Brain Struct Funct. 212:403–416. DOI: 10.1007/s00429-007-0169-6. PMID: 18200448. PMCID: PMC2226080. PMID: https://www.scopus.com/inward/record.uri?partnerID=HzOxMe3b&scp=38549090692&origin=inward.
12. Hock E, McBride S, Gnezda MT. 1989; Maternal separation anxiety: mother-infant separation from the maternal perspective. Child Dev. 60:793–802. https://psycnet.apa.org/record/1989-38627-001. DOI: 10.2307/1131019.
13. Nuss P. 2015; Anxiety disorders and GABA neurotransmission: a disturbance of modulation. Neuropsychiatr Dis Treat. 11:165–175. DOI: 10.2147/NDT.S58841. PMID: 25653526. PMCID: PMC4303399. PMID: https://www.scopus.com/inward/record.uri?partnerID=HzOxMe3b&scp=84921513295&origin=inward.
14. Lye TC, Piguet O, Grayson DA, Creasey H, Ridley LJ, Bennett HP, Broe GA. 2004; Hippocampal size and memory function in the ninth and tenth decades of life: the Sydney Older Persons Study. J Neurol Neurosurg Psychiatry. 75:548–554. DOI: 10.1136/jnnp.2003.010223. PMID: 15026494. PMCID: PMC1739036. PMID: https://www.scopus.com/inward/record.uri?partnerID=HzOxMe3b&scp=1642523794&origin=inward.
15. Heun R, Mazanek M, Atzor KR, Tintera J, Gawehn J, Burkart M, Gänsicke M, Falkai P, Stoeter P. 1997; Amygdala-hippocampal atrophy and memory performance in dementia of Alzheimer type. Dement Geriatr Cogn Disord. 8:329–336. DOI: 10.1159/000106651. PMID: 9370084. PMID: https://www.scopus.com/inward/record.uri?partnerID=HzOxMe3b&scp=0030698742&origin=inward.
16. Lee AC, Buckley MJ, Gaffan D, Emery T, Hodges JR, Graham KS. 2006; Differentiating the roles of the hippocampus and perirhinal cortex in processes beyond long-term declarative memory: a double dissociation in dementia. J Neurosci. 26:5198–5203. DOI: 10.1523/JNEUROSCI.3157-05.2006. PMID: 16687511. PMCID: PMC6674247. PMID: https://www.scopus.com/inward/record.uri?partnerID=HzOxMe3b&scp=33646926960&origin=inward.
17. Graham KS, Hodges JR. 1997; Differentiating the roles of the hippocampal complex and the neocortex in long-term memory storage: evidence from the study of semantic dementia and Alzheimer's disease. Neuropsychology. 11:77–89. DOI: 10.1037/0894-4105.11.1.77. PMID: 9055272. PMID: https://www.scopus.com/inward/record.uri?partnerID=HzOxMe3b&scp=0031027495&origin=inward.
18. O'Leary OF, Cryan JF. 2014; A ventral view on antidepressant action: roles for adult hippocampal neurogenesis along the dorsoventral axis. Trends Pharmacol Sci. 35:675–687. DOI: 10.1016/j.tips.2014.09.011. PMID: 25455365. PMID: https://www.scopus.com/inward/record.uri?partnerID=HzOxMe3b&scp=84912531587&origin=inward.
19. Goodman AM, Wheelock MD, Harnett NG, Mrug S, Granger DA, Knight DC. 2016; The hippocampal response to psychosocial stress varies with salivary uric acid level. Neuroscience. 339:396–401. DOI: 10.1016/j.neuroscience.2016.10.002. PMID: 27725214. PMCID: PMC5118067. PMID: https://www.scopus.com/inward/record.uri?partnerID=HzOxMe3b&scp=84994037287&origin=inward.
20. Kim EJ, Pellman B, Kim JJ. 2015; Stress effects on the hippocampus: a critical review. Learn Mem. 22:411–416. DOI: 10.1101/lm.037291.114. PMID: 26286651. PMCID: PMC4561403. PMID: https://www.scopus.com/inward/record.uri?partnerID=HzOxMe3b&scp=84942100658&origin=inward.
21. Aust S, Stasch J, Jentschke S, Alkan Härtwig E, Koelsch S, Heuser I, Bajbouj M. 2014; Differential effects of early life stress on hippocampus and amygdala volume as a function of emotional abilities. Hippocampus. 24:1094–1101. DOI: 10.1002/hipo.22293. PMID: 24753197. PMID: https://www.scopus.com/inward/record.uri?partnerID=HzOxMe3b&scp=84906789700&origin=inward.
22. Mohlenhoff BS, Chao LL, Buckley ST, Weiner MW, Neylan TC. 2014; Are hippocampal size differences in posttraumatic stress disorder mediated by sleep pathology? Alzheimers Dement. 10(3 Suppl):S146–S154. DOI: 10.1016/j.jalz.2014.04.016. PMID: 24924666. PMCID: PMC4111235. PMID: https://www.scopus.com/inward/record.uri?partnerID=HzOxMe3b&scp=84902457708&origin=inward.
23. Syed SA, Nemeroff CB. 2017; Early life stress, mood, and anxiety disorders. Chronic Stress (Thousand Oaks). 1:2470547017694461. DOI: 10.1177/2470547017694461. PMID: 28649671. PMCID: PMC5482282. PMID: https://www.scopus.com/inward/record.uri?partnerID=HzOxMe3b&scp=85096216020&origin=inward.
24. Xie H, Claycomb Erwin M, Elhai JD, Wall JT, Tamburrino MB, Brickman KR, Kaminski B, McLean SA, Liberzon I, Wang X. 2018; Relationship of hippocampal volumes and posttraumatic stress disorder symptoms over early posttrauma periods. Biol Psychiatry Cogn Neurosci Neuroimaging. 3:968–975. DOI: 10.1016/j.bpsc.2017.11.010. PMID: 30409391. PMCID: PMC6233727. PMID: https://www.scopus.com/inward/record.uri?partnerID=HzOxMe3b&scp=85040252331&origin=inward.
25. Shin LM, Shin PS, Heckers S, Krangel TS, Macklin ML, Orr SP, Lasko N, Segal E, Makris N, Richert K, Levering J, Schacter DL, Alpert NM, Fischman AJ, Pitman RK, Rauch SL. 2004; Hippocampal function in posttraumatic stress disorder. Hippocampus. 14:292–300. DOI: 10.1002/hipo.10183. PMID: 15132428. PMID: https://www.scopus.com/inward/record.uri?partnerID=HzOxMe3b&scp=2342576835&origin=inward.
26. Averill CL, Satodiya RM, Scott JC, Wrocklage KM, Schweinsburg B, Averill LA, Akiki TJ, Amoroso T, Southwick SM, Krystal JH, Abdallah CG. 2017; Posttraumatic stress disorder and depression symptom severities are differentially associated with hippocampal subfield volume loss in combat veterans. Chronic Stress (Thousand Oaks). 1:2470547017744538. DOI: 10.1177/2470547017744538. PMID: 29520395. PMCID: PMC5839647. PMID: https://www.scopus.com/inward/record.uri?partnerID=HzOxMe3b&scp=85068793685&origin=inward.
27. Jung MW, Wiener SI, McNaughton BL. 1994; Comparison of spatial firing characteristics of units in dorsal and ventral hippocampus of the rat. J Neurosci. 14:7347–7356. DOI: 10.1523/JNEUROSCI.14-12-07347.1994. PMID: 7996180. PMCID: PMC6576902. PMID: https://www.scopus.com/inward/record.uri?partnerID=HzOxMe3b&scp=0027973505&origin=inward.
28. Hock BJ Jr, Bunsey MD. 1998; Differential effects of dorsal and ventral hippocampal lesions. J Neurosci. 18:7027–7032. DOI: 10.1523/JNEUROSCI.18-17-07027.1998. PMID: 9712671. PMCID: PMC6792982. PMID: https://www.scopus.com/inward/record.uri?partnerID=HzOxMe3b&scp=17344371189&origin=inward.
29. Royer S, Sirota A, Patel J, Buzsáki G. 2010; Distinct representations and theta dynamics in dorsal and ventral hippocampus. J Neurosci. 30:1777–1787. DOI: 10.1523/JNEUROSCI.4681-09.2010. PMID: 20130187. PMCID: PMC2825159. PMID: https://www.scopus.com/inward/record.uri?partnerID=HzOxMe3b&scp=76149123572&origin=inward.
30. Lee AR, Kim JH, Cho E, Kim M, Park M. 2017; Dorsal and ventral hippocampus differentiate in functional pathways and differentially associate with neurological disease-related genes during postnatal development. Front Mol Neurosci. 10:331. DOI: 10.3389/fnmol.2017.00331. PMID: 29085281. PMCID: PMC5650623. PMID: https://www.scopus.com/inward/record.uri?partnerID=HzOxMe3b&scp=85032305631&origin=inward.
31. Veenema AH, Blume A, Niederle D, Buwalda B, Neumann ID. 2006; Effects of early life stress on adult male aggression and hypothalamic vasopressin and serotonin. Eur J Neurosci. 24:1711–1720. DOI: 10.1111/j.1460-9568.2006.05045.x. PMID: 17004935. PMID: https://www.scopus.com/inward/record.uri?partnerID=HzOxMe3b&scp=33748892141&origin=inward.
32. Veenema AH, Bredewold R, Neumann ID. 2007; Opposite effects of maternal separation on intermale and maternal aggression in C57BL/6 mice: link to hypothalamic vasopressin and oxytocin immunoreactivity. Psychoneuroendocrinology. 32:437–450. DOI: 10.1016/j.psyneuen.2007.02.008. PMID: 17433558. PMID: https://www.scopus.com/inward/record.uri?partnerID=HzOxMe3b&scp=34248577678&origin=inward.
33. Shiah IS, Yatham LN. 1998; GABA function in mood disorders: an update and critical review. Life Sci. 63:1289–1303. DOI: 10.1016/S0024-3205(98)00241-0. PMID: 9768867. PMID: https://www.scopus.com/inward/record.uri?partnerID=HzOxMe3b&scp=0032483234&origin=inward.
34. Kelsom C, Lu W. 2013; Development and specification of GABAergic cortical interneurons. Cell Biosci. 3:19. DOI: 10.1186/2045-3701-3-19. PMID: 23618463. PMCID: PMC3668182. PMID: https://www.scopus.com/inward/record.uri?partnerID=HzOxMe3b&scp=84878470649&origin=inward.
35. Herman JP, Ostrander MM, Mueller NK, Figueiredo H. 2005; Limbic system mechanisms of stress regulation: hypothalamo-pituitary-adrenocortical axis. Prog Neuropsychopharmacol Biol Psychiatry. 29:1201–1213. DOI: 10.1016/j.pnpbp.2005.08.006. PMID: 16271821. PMID: https://www.scopus.com/inward/record.uri?partnerID=HzOxMe3b&scp=27944442385&origin=inward.
36. Donato F, Rompani SB, Caroni P. 2013; Parvalbumin-expressing basket-cell network plasticity induced by experience regulates adult learning. Nature. 504:272–276. DOI: 10.1038/nature12866. PMID: 24336286. PMID: https://www.scopus.com/inward/record.uri?partnerID=HzOxMe3b&scp=84890343407&origin=inward.
37. Courtin J, Chaudun F, Rozeske RR, Karalis N, Gonzalez-Campo C, Wurtz H, Abdi A, Baufreton J, Bienvenu TC, Herry C. 2014; Prefrontal parvalbumin interneurons shape neuronal activity to drive fear expression. Nature. 505:92–96. DOI: 10.1038/nature12755. PMID: 24256726. PMID: https://www.scopus.com/inward/record.uri?partnerID=HzOxMe3b&scp=84892372575&origin=inward.
38. Klausberger T, Marton LF, O'Neill J, Huck JH, Dalezios Y, Fuentealba P, Suen WY, Papp E, Kaneko T, Watanabe M, Csicsvari J, Somogyi P. 2005; Complementary roles of cholecystokinin- and parvalbumin-expressing GABAergic neurons in hippocampal network oscillations. J Neurosci. 25:9782–9793. DOI: 10.1523/JNEUROSCI.3269-05.2005. PMID: 16237182. PMCID: PMC6725722. PMID: https://www.scopus.com/inward/record.uri?partnerID=HzOxMe3b&scp=27144440359&origin=inward.
39. Wu C, Sun D. 2015; GABA receptors in brain development, function, and injury. Metab Brain Dis. 30:367–379. DOI: 10.1007/s11011-014-9560-1. PMID: 24820774. PMCID: PMC4231020. PMID: https://www.scopus.com/inward/record.uri?partnerID=HzOxMe3b&scp=84939873845&origin=inward.
40. Cenquizca LA, Swanson LW. 2007; Spatial organization of direct hippocampal field CA1 axonal projections to the rest of the cerebral cortex. Brain Res Rev. 56:1–26. DOI: 10.1016/j.brainresrev.2007.05.002. PMID: 17559940. PMCID: PMC2171036. PMID: https://www.scopus.com/inward/record.uri?partnerID=HzOxMe3b&scp=36049036839&origin=inward.
41. Loureiro M, Kramar C, Renard J, Rosen LG, Laviolette SR. 2016; Cannabinoid transmission in the hippocampus activates nucleus accumbens neurons and modulates reward and aversion-related emotional salience. Biol Psychiatry. 80:216–225. DOI: 10.1016/j.biopsych.2015.10.016. PMID: 26681496. PMID: https://www.scopus.com/inward/record.uri?partnerID=HzOxMe3b&scp=84949659520&origin=inward.
42. Swanson LW. 1981; A direct projection from Ammon's horn to prefrontal cortex in the rat. Brain Res. 217:150–154. DOI: 10.1016/0006-8993(81)90192-X. PMID: 7260612. PMID: https://www.scopus.com/inward/record.uri?partnerID=HzOxMe3b&scp=0019407810&origin=inward.
43. Tannenholz L, Jimenez JC, Kheirbek MA. 2014; Local and regional heterogeneity underlying hippocampal modulation of cognition and mood. Front Behav Neurosci. 8:147. DOI: 10.3389/fnbeh.2014.00147. PMID: 24834033. PMCID: PMC4018538. PMID: https://www.scopus.com/inward/record.uri?partnerID=HzOxMe3b&scp=84899815131&origin=inward.
44. Chang CH, Gean PW. 2019; The ventral hippocampus controls stress-provoked impulsive aggression through the ventromedial hypothalamus in post-weaning social isolation mice. Cell Rep. 28:1195–1205.e3. DOI: 10.1016/j.celrep.2019.07.005. PMID: 31365864. PMID: https://www.scopus.com/inward/record.uri?partnerID=HzOxMe3b&scp=85069592810&origin=inward.
45. Herman JP, Mueller NK, Figueiredo H. 2004; Role of GABA and glutamate circuitry in hypothalamo-pituitary-adrenocortical stress integration. Ann N Y Acad Sci. 1018:35–45. DOI: 10.1196/annals.1296.004. PMID: 15240350. PMID: https://www.scopus.com/inward/record.uri?partnerID=HzOxMe3b&scp=3242703975&origin=inward.
46. Jacobson L, Sapolsky R. 1991; The role of the hippocampus in feedback regulation of the hypothalamic-pituitary-adrenocortical axis. . Endocr Rev. 12:118–134. DOI: 10.1210/edrv-12-2-118. PMID: 2070776. PMID: https://www.scopus.com/inward/record.uri?partnerID=HzOxMe3b&scp=0025804787&origin=inward.
47. Shin SY, Han SH, Woo RS, Jang SH, Min SS. 2016; Adolescent mice show anxiety- and aggressive-like behavior and the reduction of long-term potentiation in mossy fiber-CA3 synapses after neonatal maternal separation. Neuroscience. 316:221–231. DOI: 10.1016/j.neuroscience.2015.12.041. PMID: 26733385. PMID: https://www.scopus.com/inward/record.uri?partnerID=HzOxMe3b&scp=84953231951&origin=inward.
48. Shin SY, Baek NJ, Han SH, Min SS. 2019; Chronic administration of ketamine ameliorates the anxiety- and aggressive-like behavior in adolescent mice induced by neonatal maternal separation. Korean J Physiol Pharmacol. 23:81–87. DOI: 10.4196/kjpp.2019.23.1.81. PMID: 30627013. PMCID: PMC6315094. PMID: https://www.scopus.com/inward/record.uri?partnerID=HzOxMe3b&scp=85060146333&origin=inward.
49. Liu D, Caldji C, Sharma S, Plotsky PM, Meaney MJ. 2000; Influence of neonatal rearing conditions on stress-induced adrenocorticotropin responses and norepinepherine release in the hypothalamic paraventricular nucleus. J Neuroendocrinol. 12:5–12. DOI: 10.1046/j.1365-2826.2000.00422.x. PMID: 10692138. PMID: https://www.scopus.com/inward/record.uri?partnerID=HzOxMe3b&scp=0033952402&origin=inward.
50. Andersen SL, Teicher MH. 2004; Delayed effects of early stress on hippocampal development. Neuropsychopharmacology. 29:1988–1993. DOI: 10.1038/sj.npp.1300528. PMID: 15316569. PMID: https://www.scopus.com/inward/record.uri?partnerID=HzOxMe3b&scp=4544304901&origin=inward.
51. Borel C, Welti DH, Fernandez I, Colmenares M. 1993; Dicranin, an antimicrobial and 15-lipoxygenase inhibitor from the moss Dicranum scoparium. J Nat Prod. 56:1071–1077. DOI: 10.1021/np50097a010. PMID: 8377015. PMID: https://www.scopus.com/inward/record.uri?partnerID=HzOxMe3b&scp=0027185686&origin=inward.
52. Li A, Zhang RX, Wang Y, Zhang H, Ren K, Berman BM, Tan M, Lao L. 2007; Corticosterone mediates electroacupuncture-produced anti-edema in a rat model of inflammation. BMC Complement Altern Med. 7:27. DOI: 10.1186/1472-6882-7-27. PMID: 17697336. PMCID: PMC1976320. PMID: https://www.scopus.com/inward/record.uri?partnerID=HzOxMe3b&scp=34548723303&origin=inward.
53. Goymann W, Möstl E, Gwinner E. 2002; Corticosterone metabolites can be measured noninvasively in excreta of European Stonechats (Saxicola torquata rubicola). The Auk. 119:1167–1173. https://academic.oup.com/auk/article/119/4/1167/5561800. DOI: 10.1642/0004-8038(2002)119[1167:CMCBMN]2.0.CO;2.
54. Millspaugh JJ, Washburn BE, Milanick MA, Slotow R, van Dyk G. 2003; Effects of heat and chemical treatments on fecal glucocorticoid measurements: implications for sample transport. Wildlife Soc Bull. 31:399–406. https://www.jstor.org/stable/3784319.
55. Vázquez-Palacios G, Retana-Márquez S, Bonilla-Jaime H, Velázquez-Moctezuma J. 2001; Further definition of the effect of corticosterone on the sleep-wake pattern in the male rat. Pharmacol Biochem Behav. 70:305–310. DOI: 10.1016/S0091-3057(01)00620-7. PMID: 11701201. PMID: https://www.scopus.com/inward/record.uri?partnerID=HzOxMe3b&scp=0035169314&origin=inward.
56. Paxinos G, Watson C. 2013. The rat brain in stereotaxic coordinates. Elsevier Science;Amsterdam: https://www.elsevier.com/books/the-rat-brain-in-stereotaxic-coordinates/paxinos/978-0-12-391949-6.
57. Witter MP, Amaral DG. Paxinos G, editor. 2004. Hippocampal formation. The rat nervous system. Elsevier Inc.;Amsterdam: p. 635–704. https://doi.org/10.1016/B978-012547638-6/50022-5. DOI: 10.1016/B978-012547638-6/50022-5. PMID: https://www.scopus.com/inward/record.uri?partnerID=HzOxMe3b&scp=84942605875&origin=inward.
58. Lajud N, Torner L. 2015; Early life stress and hippocampal neurogenesis in the neonate: sexual dimorphism, long term consequences and possible mediators. Front Mol Neurosci. 8:3. DOI: 10.3389/fnmol.2015.00003. PMID: 25741234. PMCID: PMC4327304. PMID: https://www.scopus.com/inward/record.uri?partnerID=HzOxMe3b&scp=84923309306&origin=inward.
59. Litvin Y, Tovote P, Pentkowski NS, Zeyda T, King LB, Vasconcellos AJ, Dunlap C, Spiess J, Blanchard DC, Blanchard RJ. 2010; Maternal separation modulates short-term behavioral and physiological indices of the stress response. Horm Behav. 58:241–249. DOI: 10.1016/j.yhbeh.2010.03.010. PMID: 20298695. PMID: https://www.scopus.com/inward/record.uri?partnerID=HzOxMe3b&scp=77953360240&origin=inward.
60. He T, Guo C, Wang C, Hu C, Chen H. 2020; Effect of early life stress on anxiety and depressive behaviors in adolescent mice. Brain Behav. 10:e01526. DOI: 10.1002/brb3.1526. PMID: 31961515. PMCID: PMC7066350. PMID: 1d97728c73c84648a64b209073c8af64. PMID: https://www.scopus.com/inward/record.uri?partnerID=HzOxMe3b&scp=85078719618&origin=inward.
61. Ennaceur A. 2012; Open space anxiety test in rodents: the elevated platform with steep slopes. Methods Mol Biol. 829:177–191. DOI: 10.1007/978-1-61779-458-2_11. PMID: 22231814. PMID: https://www.scopus.com/inward/record.uri?partnerID=HzOxMe3b&scp=84856329943&origin=inward.
62. Koolhaas JM, Coppens CM, de Boer SF, Buwalda B, Meerlo P, Timmermans PJ. 2013; The resident-intruder paradigm: a standardized test for aggression, violence and social stress. J Vis Exp. (77):e4367. DOI: 10.3791/4367. PMID: 23852258. PMCID: PMC3731199. PMID: https://www.scopus.com/inward/record.uri?partnerID=HzOxMe3b&scp=84894672265&origin=inward.
63. Kikusui T, Mori Y. 2009; Behavioural and neurochemical consequences of early weaning in rodents. J Neuroendocrinol. 21:427–431. DOI: 10.1111/j.1365-2826.2009.01837.x. PMID: 19207810. PMID: https://www.scopus.com/inward/record.uri?partnerID=HzOxMe3b&scp=63549145538&origin=inward.
64. Ito A, Kikusui T, Takeuchi Y, Mori Y. 2006; Effects of early weaning on anxiety and autonomic responses to stress in rats. Behav Brain Res. 171:87–93. DOI: 10.1016/j.bbr.2006.03.023. PMID: 16677722. PMID: https://www.scopus.com/inward/record.uri?partnerID=HzOxMe3b&scp=33646540405&origin=inward.
65. Millstein RA, Holmes A. 2007; Effects of repeated maternal separation on anxiety- and depression-related phenotypes in different mouse strains. Neurosci Biobehav Rev. 31:3–17. DOI: 10.1016/j.neubiorev.2006.05.003. PMID: 16950513. PMID: https://www.scopus.com/inward/record.uri?partnerID=HzOxMe3b&scp=33845342923&origin=inward.
66. Vetulani J. 2013; Early maternal separation: a rodent model of depression and a prevailing human condition. Pharmacol Rep. 65:1451–1461. DOI: 10.1016/S1734-1140(13)71505-6. PMID: 24552992. PMID: https://www.scopus.com/inward/record.uri?partnerID=HzOxMe3b&scp=84886668177&origin=inward.
67. Bian Y, Yang L, Wang Z, Wang Q, Zeng L, Xu G. 2015; Repeated three-hour maternal separation induces depression-like behavior and affects the expression of hippocampal plasticity-related proteins in C57BL/6N mice. Neural Plast. 2015:627837. DOI: 10.1155/2015/627837. PMID: 26798520. PMCID: PMC4700195. PMID: https://www.scopus.com/inward/record.uri?partnerID=HzOxMe3b&scp=84983094590&origin=inward.
68. Zheng Y, He J, Guo L, Yao L, Zheng X, Yang Z, Xia Y, Wu X, Su Y, Xu N, Chen Y. 2019; Transcriptome analysis on maternal separation rats with depression-related manifestations ameliorated by electroacupuncture. Front Neurosci. 13:314. DOI: 10.3389/fnins.2019.00314. PMID: 31024237. PMCID: PMC6460510. PMID: https://www.scopus.com/inward/record.uri?partnerID=HzOxMe3b&scp=85068475069&origin=inward.
69. Žiburkus J, Cressman JR, Schiff SJ. 2013; Seizures as imbalanced up states: excitatory and inhibitory conductances during seizure-like events. J Neurophysiol. 109:1296–1306. DOI: 10.1152/jn.00232.2012. PMID: 23221405. PMCID: PMC3602838. PMID: https://www.scopus.com/inward/record.uri?partnerID=HzOxMe3b&scp=84874619418&origin=inward.
70. Gao R, Penzes P. 2015; Common mechanisms of excitatory and inhibitory imbalance in schizophrenia and autism spectrum disorders. Curr Mol Med. 15:146–167. DOI: 10.2174/1566524015666150303003028. PMID: 25732149. PMCID: PMC4721588. PMID: https://www.scopus.com/inward/record.uri?partnerID=HzOxMe3b&scp=84930196553&origin=inward.
71. Bi D, Wen L, Wu Z, Shen Y. 2020; GABAergic dysfunction in excitatory and inhibitory (E/I) imbalance drives the pathogenesis of Alzheimer's disease. Alzheimers Dement. 16:1312–1329. DOI: 10.1002/alz.12088. PMID: 32543726. PMID: https://www.scopus.com/inward/record.uri?partnerID=HzOxMe3b&scp=85091958888&origin=inward.
72. Nelson SB, Valakh V. 2015; Excitatory/inhibitory balance and circuit homeostasis in autism spectrum disorders. Neuron. 87:684–698. DOI: 10.1016/j.neuron.2015.07.033. PMID: 26291155. PMCID: PMC4567857. PMID: https://www.scopus.com/inward/record.uri?partnerID=HzOxMe3b&scp=84939540039&origin=inward.
73. Ruden JB, Dugan LL, Konradi C. 2021; Parvalbumin interneuron vulnerability and brain disorders. Neuropsychopharmacology. 46:279–287. DOI: 10.1038/s41386-020-0778-9. PMID: 32722660. PMCID: PMC7852528. PMID: https://www.scopus.com/inward/record.uri?partnerID=HzOxMe3b&scp=85088704526&origin=inward.
74. Orsini CA, Kim JH, Knapska E, Maren S. 2011; Hippocampal and prefrontal projections to the basal amygdala mediate contextual regulation of fear after extinction. J Neurosci. 31:17269–17277. DOI: 10.1523/JNEUROSCI.4095-11.2011. PMID: 22114293. PMCID: PMC3241946. PMID: https://www.scopus.com/inward/record.uri?partnerID=HzOxMe3b&scp=81855184631&origin=inward.
75. Santos M, D'Amico D, Spadoni O, Amador-Arjona A, Stork O, Dierssen M. 2013; Hippocampal hyperexcitability underlies enhanced fear memories in TgNTRK3, a panic disorder mouse model. J Neurosci. 33:15259–15271. DOI: 10.1523/JNEUROSCI.2161-13.2013. PMID: 24048855. PMCID: PMC6618414.
76. Mahmoodkhani M, Ghasemi M, Derafshpour L, Amini M, Mehranfard N. 2020; Long-term decreases in the expression of calcineurin and GABAA receptors induced by early maternal separation are associated with increased anxiety-like behavior in adult male rats. Dev Neurosci. 42:135–144. DOI: 10.1159/000512221. PMID: 33341802.
77. Herman JP, Mueller NK. 2006; Role of the ventral subiculum in stress integration. Behav Brain Res. 174:215–224. DOI: 10.1016/j.bbr.2006.05.035. PMID: 16876265.
78. Dedovic K, Duchesne A, Andrews J, Engert V, Pruessner JC. 2009; The brain and the stress axis: the neural correlates of cortisol regulation in response to stress. Neuroimage. 47:864–871. DOI: 10.1016/j.neuroimage.2009.05.074. PMID: 19500680.
79. Parfitt GM, Nguyen R, Bang JY, Aqrabawi AJ, Tran MM, Seo DK, Richards BA, Kim JC. 2017; Bidirectional control of anxiety-related behaviors in mice: role of inputs arising from the ventral hippocampus to the lateral septum and medial prefrontal cortex. Neuropsychopharmacology. 42:1715–1728. DOI: 10.1038/npp.2017.56. PMID: 28294135. PMCID: PMC5518909.
80. Jimenez JC, Su K, Goldberg AR, Luna VM, Biane JS, Ordek G, Zhou P, Ong SK, Wright MA, Zweifel L, Paninski L, Hen R, Kheirbek MA. 2018; Anxiety cells in a hippocampal-hypothalamic circuit. Neuron. 97:670–683.e6. DOI: 10.1016/j.neuron.2018.01.016. PMID: 29397273. PMCID: PMC5877404.
81. Bernier BE, Lacagnina AF, Ayoub A, Shue F, Zemelman BV, Krasne FB, Drew MR. 2017; Dentate gyrus contributes to retrieval as well as encoding: evidence from context fear conditioning, recall, and extinction. J Neurosci. 37:6359–6371. DOI: 10.1523/JNEUROSCI.3029-16.2017. PMID: 28546308. PMCID: PMC5490069.
82. Karolewicz B, Maciag D, O'Dwyer G, Stockmeier CA, Feyissa AM, Rajkowska G. 2010; Reduced level of glutamic acid decarboxylase-67 kDa in the prefrontal cortex in major depression. Int J Neuropsychopharmacol. 13:411–420. DOI: 10.1017/S1461145709990587. PMID: 20236554. PMCID: PMC2857696.
83. Wang J, Tang J, Liang X, Luo Y, Zhu P, Li Y, Xiao K, Jiang L, Yang H, Xie Y, Zhang L, Deng Y, Li J, Tang Y. 2021; Hippocampal PGC-1α-mediated positive effects on parvalbumin interneurons are required for the antidepressant effects of running exercise. Transl Psychiatry. 11:222. DOI: 10.1038/s41398-021-01339-1. PMID: 33859158. PMCID: PMC8050070. PMID: 94fef0a417fc4695a7aba104a91ccfd8.
84. Czéh B, Varga ZK, Henningsen K, Kovács GL, Miseta A, Wiborg O. 2015; Chronic stress reduces the number of GABAergic interneurons in the adult rat hippocampus, dorsal-ventral and region-specific differences. Hippocampus. 25:393–405. DOI: 10.1002/hipo.22382. PMID: 25331166.
85. Csabai D, Seress L, Varga Z, Ábrahám H, Miseta A, Wiborg O, Czéh B. 2017; Electron microscopic analysis of hippocampal axo-somatic synapses in a chronic stress model for depression. Hippocampus. 27:17–27. DOI: 10.1002/hipo.22650. PMID: 27571571. PMCID: PMC5215622.
86. Joëls M, Karst H, Krugers HJ, Lucassen PJ. 2007; Chronic stress: implications for neuronal morphology, function and neurogenesis. Front Neuroendocrinol. 28:72–96. DOI: 10.1016/j.yfrne.2007.04.001. PMID: 17544065.
87. Travis S, Coupland NJ, Silversone PH, Huang Y, Fujiwara E, Carter R, Seres P, Malykhin NV. 2015; Dentate gyrus volume and memory performance in major depressive disorder. J Affect Disord. 172:159–164. DOI: 10.1016/j.jad.2014.09.048. PMID: 25451411.
88. Deller T, Haas CA, Deissenrieder K, Del Turco D, Coulin C, Gebhardt C, Drakew A, Schwarz K, Mundel P, Frotscher M. 2002; Laminar distribution of synaptopodin in normal and reeler mouse brain depends on the position of spine-bearing neurons. J Comp Neurol. 453:33–44. DOI: 10.1002/cne.10362. PMID: 12357430.
89. Ulrich-Lai YM, Herman JP. 2009; Neural regulation of endocrine and autonomic stress responses. Nat Rev Neurosci. 10:397–409. DOI: 10.1038/nrn2647. PMID: 19469025. PMCID: PMC4240627.
90. Smith SM, Vale WW. 2006; The role of the hypothalamic-pituitary-adrenal axis in neuroendocrine responses to stress. Dialogues Clin Neurosci. 8:383–395. DOI: 10.31887/DCNS.2006.8.4/ssmith. PMID: 17290797. PMCID: PMC3181830.
91. Stephens MA, Wand G. 2012; Stress and the HPA axis: role of glucocorticoids in alcohol dependence. Alcohol Res. 34:468–483. PMID: 23584113. PMCID: PMC3860380.
92. Sapolsky RM. 2000; Glucocorticoids and hippocampal atrophy in neuropsychiatric disorders. Arch Gen Psychiatry. 57:925–935. DOI: 10.1001/archpsyc.57.10.925. PMID: 11015810.
93. Weeden CS, Roberts JM, Kamm AM, Kesner RP. 2015; The role of the ventral dentate gyrus in anxiety-based behaviors. Neurobiol Learn Mem. 118:143–149. DOI: 10.1016/j.nlm.2014.12.002. PMID: 25498221.
94. Lesuis SL, Brosens N, Immerzeel N, van der Loo RJ, Mitrić M, Bielefeld P, Fitzsimons CP, Lucassen PJ, Kushner SA, van den Oever MC, Krugers HJ. 2021; Glucocorticoids promote fear generalization by increasing the size of a dentate gyrus engram cell population. Biol Psychiatry. 90:494–504. DOI: 10.1016/j.biopsych.2021.04.010. PMID: 34503674.
95. Zetzsche T, Preuss UW, Frodl T, Schmitt G, Seifert D, Münchhausen E, Tabrizi S, Leinsinger G, Born C, Reiser M, Möller HJ, Meisenzahl EM. 2007; Hippocampal volume reduction and history of aggressive behaviour in patients with borderline personality disorder. Psychiatry Res. 154:157–170. DOI: 10.1016/j.pscychresns.2006.05.010. PMID: 17306512.
96. Davies CH, Starkey SJ, Pozza MF, Collingridge GL. 1991; GABA autoreceptors regulate the induction of LTP. Nature. 349:609–611. DOI: 10.1038/349609a0. PMID: 1847993.
97. Lussier SJ, Stevens HE. 2016; Delays in GABAergic interneuron development and behavioral inhibition after prenatal stress. Dev Neurobiol. 76:1078–1091. DOI: 10.1002/dneu.22376. PMID: 26724783. PMCID: PMC6355144.
Fig. 1
Experimental design.
To characterize the effect of MS, pups were weaned at PND 21 and regrouped by gender at PND 22. The behavior changes by MS in the adolescence period were analyzed using an EPM test and RI test. EPM test was conducted between PND 42 and PND 46. RI test was conducted at PND 49 following single-housing of animals for two weeks from PND 35. CORT assay and IHC for GAD67 and PV experiments were conducted between PND 42 and 49. MS, maternal separation; PND, postnatal day; EPM, elevated plus maze; RI, resident-intruder; CORT, corticosterone; IHC, immunohistochemistry; GAD, glutamic acid decarboxylase; PV, parvalbumin.
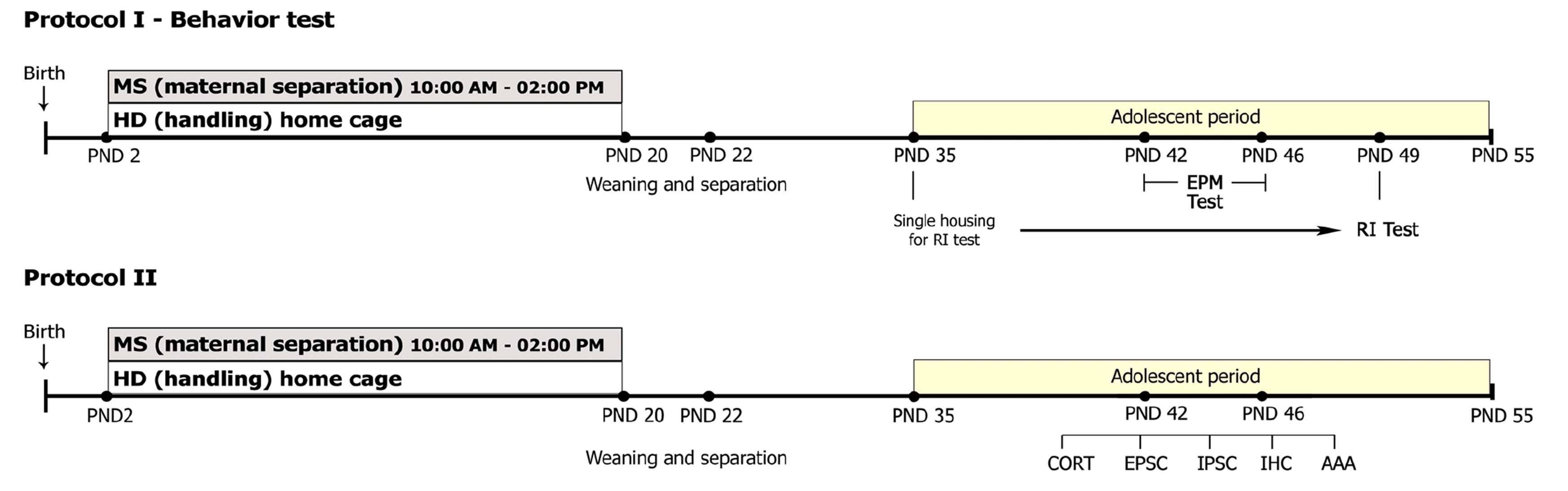
Fig. 2
Assessment of anxiety and aggressive behavior caused by neonatal maternal separation (MS).
EPM: (A, B) times spent in the open arms (A) and closed arms (B): times to open arms or closed arms/total time spent. (C, D) the ratio of entries into the open and the closed arms of total entries: the ratio of entries into the open arms (C) or the closed arms (D). RI test. (E) Latency to attack: time between the introduction of the intruder and the first attack of the resident. (F) Duration of attack: total time spent in clinch attack, lateral threat, and keep down. (G) The total number of attacks by the resident. (H) Corticosterone levels in HD and MS mice. Plasma corticosterone levels in mice (EPM: n = 9; RI test: n = 10; CORT: n = 10). EPM, elevated plus maze; RI, resident-intruder; HD, handling; CORT, corticosterone. Values are expressed as the mean ± SEM. Student’s t-test. *p < 0.05, **p < 0.01, ***p < 0.001.
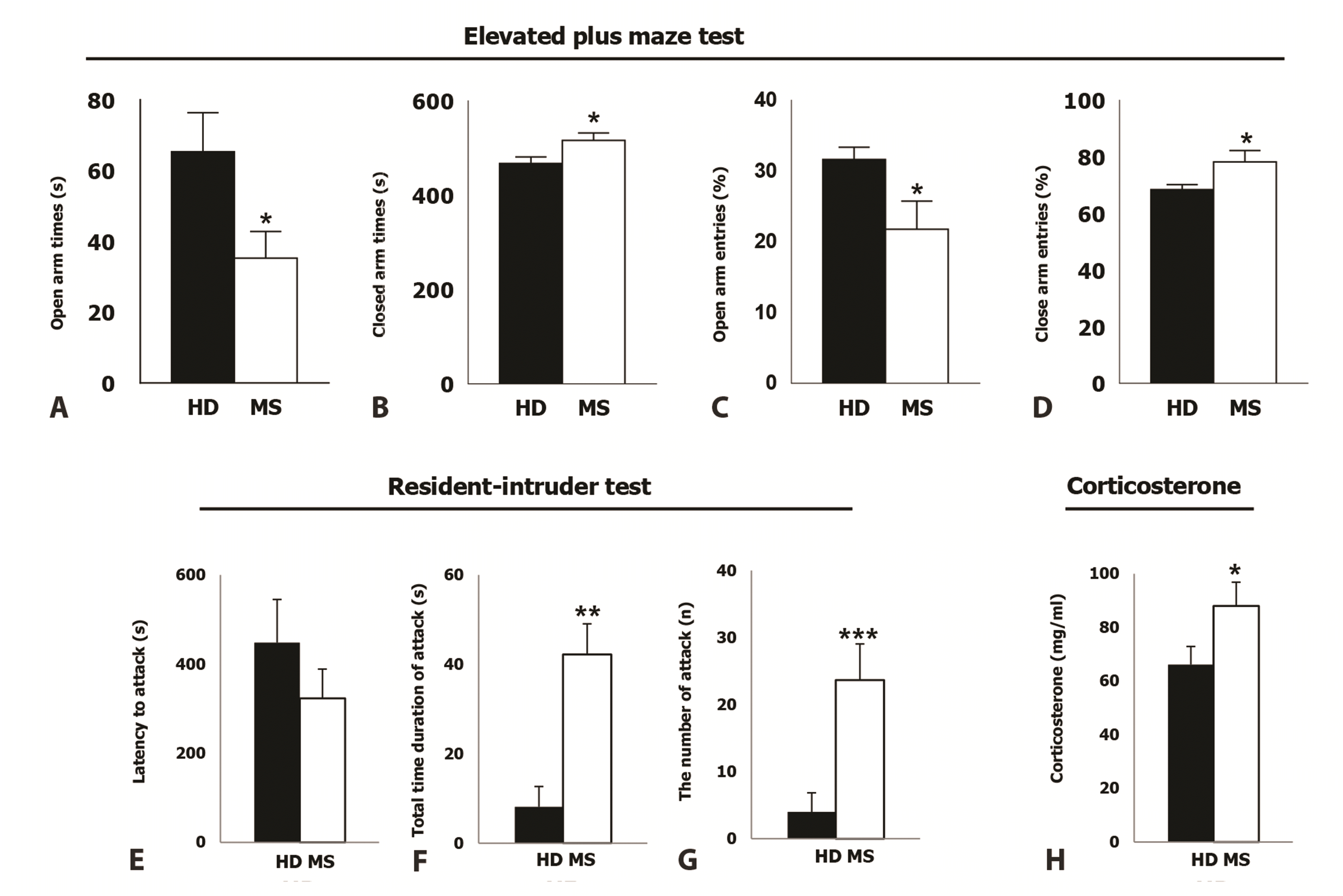
Fig. 3
Glutamic acid decarboxylase 67 (GAD67).
Hippocampal distribution of GAD67-ir interneurons in the various experimental mice. Representative micrographs of GAD67-stained whole hippocampal transverse sections from the hippocampal region (A, E), DG (B, F), CA3 (C, G), CA1 (D, H) of HD (A–D), MS (E–H) treated mice. The number of GAD67-ir interneurons in the entire hippocampal region (A, E), DG (B, F), CA3 (C, G), and CA1 (D, H) was higher in the MS group than in the HD group. Scale bar = 100-μm (A, E), Scale bar = 200-μm (B–D, F–H). ir, immunoreactive; DG, dentate gyrus; MS, maternal separation; HD, handling; gl, granular layer; ml, molecular layer; so, stratum oriens; sp, stratum pyramidale; sl, stratum lacunosum; sr, stratum radiatum; slm, stratum lacunosum-moleculare. Values are expressed as the means ± SEM. Student’s t-test (n = 13). **p < 0.01, ***p < 0.001.

Fig. 4
Glutamic acid decarboxylase 67 (GAD67).
Distribution in areas near the Sub of GAD67-immunoreactive interneurons in the different experimental mice. Representative micrographs of GAD67-stained whole hippocampal transverse sections from the total region near Sub (A, E), Sub (B, F), PrS (C, G), PaS (D, H) of HD (A–D), MS (E–H) treated mice. The number of GAD67-ir interneurons in the Sub (B, F), PrS (C, G) PaS (D, H) was higher in the MS group than in the HD group. Scale bar = 400-μm, x40 (A–H). MS, maternal separation; HD, handling; pyr, pyramidal; slm, stratum lacunosum-moleculare; Sub, subiculum; PrS, presubiculum; PaS, parasubiculum. Values are expressed as the means ± SEM. Student’s t-test (n = 13). *p < 0.05, **p < 0.01, ***p < 0.001.

Fig. 5
Parvalbumin (PV).
Hippocampal distribution of PV-immunoreactive interneurons in the different experimental mice. Representative micrographs of PV-stained whole hippocampal transverse sections from the hippocampal region (A, E), DG (B, F), CA3 (C, G), CA1 (D, H) of HD (A–D), MS (E–H) treated mice. The number of PV interneurons in the whole hippocampal region DG (B, F), CA3 (C, G), and CA1 (D, H) was higher in the MS group than in the HD group. Scale bar = 100-μm (A, E). Scale bar = 200-μm (B–D, F–H). DG, dentate gyrus; MS, maternal separation; HD, handling; gl, granular layer; ml, molecular layer; so, stratum oriens; sp, stratum pyramidale; sl, stratum lacunosum; sr, stratum radiatum; slm, stratum lacunosum-moleculare. Values are expressed as the means ± SEM. Student’s t-test (n = 13). *p < 0.05, **p < 0.01, ***p < 0.001.

Fig. 6
Parvalbumin (PV).
Hippocampal distribution of PV-immunoreactive interneurons in the different experimental mice. Representative micrographs of PV-stained whole hippocampal transverse sections from the total region near Sub (A, E), Sub (B, F), PrS (C, G), PaS (D, H) of HD (A–D), MS (E–H) treated mice. The number of PV interneurons in the PrS (C, G) PaS (D, H) was higher in the MS group than in the HD group. There was no significant difference in the number of PV interneurons in the Sub (B, F) between the MS and the HD groups. Scale bar = 400-μm (A–H). Sub, subiculum; PrS, presubiculum; PaS, parasubiculum; MS, maternal separation; HD, handling; pyr, pyramidal; slm, stratum lacunosum-moleculare. Values are expressed as the means ± SEM. Student’s t-test (n = 13). **p < 0.01, ***p < 0.001.
