Abstract
Background
Numerous studies suggest that intravenous propofol is superior to inhaled volatile anesthetic. This study compared the changes in the endoplasmic reticulum (ER) stress of cancer cells and lymphocytes after propofol- and sevoflurane-based anesthesia during breast cancer surgery.
Methods
We randomized 53 patients undergoing breast cancer surgery to propofol (n = 28) and sevoflurane (n = 25) anesthesia groups. Blood samples were obtained immediately before inducing anesthesia, and 1 and 24 h postoperatively. Human breast cancer cell lines were cultured and treated with patient plasma, and the frequency of C/EBP homologous protein (CHOP) on the cancer cell lines and lymphocytes was measured. The neutrophil-to-lymphocyte ratio in plasma was evaluated in both groups.
The endoplasmic reticulum (ER) synthesizes transmembrane proteins and lipids, and stores calcium [1]. Conditions such as hypoxia and inflammation impose stress on cells. They enhance ER stress to induce apoptosis for normal cellular homeostasis [2]. Numerous diseases such as multiple myeloma, neurodegenerative disease, and diabetes are associated with ER stress, and cancer is associated with alteration of ER stress [3,4].
Propofol is considered superior to sevoflurane for attenuating cancer progression compared to inhaled volatile anesthetics, although this is controversial [5–8]. The effects of propofol on cancer are derived from its anti-inflammatory properties, while sevoflurane causes immunosuppression and promotes cancer progression [7]. Propofol exerts a neuroprotective effect by reducing ER stress of normal neurons, unlike the neurotoxic volatile anesthetics that enhance ER stress [9,10]. However, few studies have compared changes in ER stress after propofol- and volatile anesthetic-based anesthesia in a cancer environment.
We hypothesized that propofol would induce greater ER stress in cancer cells than sevoflurane. The study was designed to compare the changes in ER stress of cancer cells and lymphocytes between propofol- and sevoflurane-based anesthesia in patients undergoing breast cancer surgery.
The study was approved by the Institutional Review Board (Konkuk University Medical Center, Seoul, Korea; KUH1160108) and registered at Clinicaltrials.gov (NCT03561831), and was conducted in accordance with the Helsinki Declaration-2013. Informed consent was obtained from all patients before the operation. Female Koreans with American Society of Anesthesiologists class I to II physical status, who were scheduled to undergo breast cancer surgery, were enrolled in this study. Patients were excluded based on the following criteria: age < 20 years old, abnormal preoperative laboratory results, history of cancer, history of propofol or sevoflurane allergy, or other concurrent surgery. On entering the operating room, each patient was randomly allocated to propofol-based (Propofol) or sevoflurane-based (Sevoflurane) anesthesia groups. A random-permuted block design was used for randomization. The medical team involved in the patient care was blind to the study. All data were collected by trained observers who were also blind to the study and did not participate in patient care.
The anesthesia techniques were standardized. No patient received pre-anesthetic medication. Anesthesia was induced after routine non-invasive monitoring, including the bispectral index (BIS). For the Propofol group, an initial effect-site propofol concentration of 4.0 µg/ml was administered intravenously under a modified Marsh model (ke0 1.21/min) using a target-controlled infusion (TCI) device (Orchestra® Base Primea; Fresenius Vial, France). For the Sevoflurane group, thiopental sodium (5 mg/kg) was administered intravenously to induce anesthesia. After loss of consciousness, mask ventilation was confirmed and rocuronium 0.6 mg/kg was administered intravenously. Remifentanil (5.0 ng/ml) was intravenously administered under the Minto model [11], and maintained until the end of surgery. After tracheal intubation, propofol or sevoflurane was titrated to maintain a BIS of 40 to 60. The mean systemic blood pressure was maintained within 20% of the baseline or > 60 mmHg during anesthesia. At the end of the surgery, the administration of propofol or sevoflurane with remifentanil was stopped and ketorolac 0.5 mg/kg was given intravenously for postoperative analgesia. Residual neuromuscular paralysis was antagonized with neostigmine 0.03 mg/kg and glycopyrrolate 0.008 mg/kg with neuromuscular transmission monitoring. Intravenous patient-controlled analgesia (PCA) was available on demand for patients undergoing radical mastectomies. The total PCA volume was 200 ml, consisting of fentanyl (2,000 µg; 40 ml), ramosetron (0.6 mg; 4 ml), and normal saline (156 ml). The PCA device (Gemstar Pump™; Hospira, USA) was programmed to deliver 0.03 ml/kg/h as the basal infusion rate and 0.05 ml/kg on demand, with a 15 min lock-out time. After tracheal extubation, patients were transferred to the post-anesthesia care unit (PACU). Postoperative medical treatment and decision making were under the control of the surgeon responsible, in accordance with standard institutional regimens.
Venous blood samples were collected immediately before inducing anesthesia (Preop), on arrival in the PACU (Post 1 h), and 24 h postoperatively (Post 24 h). Blood samples were obtained without or with minimal stasis (within 30 s) and centrifuged immediately at 3000 rpm for 15 min at 4°C. Plasma samples were frozen within 1 h of sampling and stored at -80°C until the analysis.
The Michigan Cancer Foundation-7 (MCF-7) human breast cancer cell line (AcceGen Biotech, USA) was cultured in Roswell Park Memorial Institute medium 1640 (RPMI 1640) supplemented with 10% fetal bovine serum and 1% penicillin. The medium was replaced every 3–5 days. Cells were subcultured using the trypsin-ethylenediaminetetraacetic acid method.
To culture the MCF-7 cell line with plasma of breast cancer patients, 15,000 cells were counted and mixed with 4 μl of breast cancer patient plasma in a total volume of 100 μl. Then they were transferred into the wells of 96-well cell culture plates. After 24 h of incubation, the cells in each well were transferred to fluorescence-activated cell sorting (FACS) tubes. Then, the cells were washed twice with FACS buffer (0.1% bovine serum albumin and 0.05% sodium azide in 1 × phosphate-buffered saline [PBS]) for 5 min. After washing, the cells were fixed with fixation buffer (BD Biosciences, USA) and washed with FACS buffer for 5 min. Then, they were incubated with the 1 × foxp3 perm buffer (BioLegend, USA) for 15 min and washed with FACS buffer for a further 5 min. The cells were stained with DNA Damage Inducible Transcript 3/CHOP rabbit anti-human polyclonal antibody (Cat No. LS-C16732; LSbio, USA) for 30 min, and washed with FACS buffer for 5 min. Then, they were stained with goat anti-Rabbit IgG (H + L) secondary antibody (Cat No. A-11034; Invitrogen, USA) and incubated for 30 min. Finally, they were washed with FACS buffer again.
Peripheral blood mononuclear cells (PBMCs) were isolated using density-gradient centrifugation for 20 min over Biocoll separating solution (Biochrom, Germany). The PBMCs were washed with 1 × PBS (pH 7.4, no calcium, no magnesium), 155.1 mM sodium chloride (NaCl), 2.9 mM sodium phosphate dibasic (Na2HPO4-7H2O), and 1.0 mM potassium phosphate monobasic (KH2PO4) solution. After isolating the lymphocytes, the cells were stained with fluorescein isothiocyanate, an anti-human cluster of differentiation 4 antibody (BD Biosciences, USA) at room temperature for 30 min in the dark. After fixation, the cells were permeabilized with FACS™ Perm2 (BD Biosciences, USA) buffer according to the manufacturer’s instructions. After permeabilization, the cells were stained and incubated with the same CHOP antibodies used in the MCF-7 cell line culture, using the same methods.
All data were collected using the BD Accuri C6 flow cytometer (BD Biosciences, USA), and then analyzed with FlowJo™ software (Tree Star, USA).
The differential leukocyte count was determined preoperatively, and at 24 h postoperatively. To calculate the neutrophil to lymphocyte ratio, the ratio of neutrophils to leukocytes was divided by the ratio of lymphocytes to leukocytes.
The maximal and minimal effect-site concentrations of propofol and the maximal and minimal end-expiratory concentrations of sevoflurane were recorded during anesthesia. Postoperative pain was assessed using a visual analogue scale (VAS) ranging from 0 mm (no pain) to 100 mm (worst pain imaginable). Intravenous ketorolac 0.5 mg/kg was administered on demand as an additional rescue analgesic. The perioperative opioid doses were recorded.
The primary outcome measure was the frequency of CHOP on MCF-7 cell lines over time in the Propofol and Sevoflurane groups. In a pilot study of 10 patients, we calculated a standardized effect size of 0.482 for the group difference in CHOP frequency on MCF-7 cell lines. For 80% power to detect intergroup differences using two-way repeated-measured analysis of variance (ANOVA) at the 5% significance level (α), a sample size of 22 per group was needed. Ultimately, 53 patients were enrolled, assuming a drop rate of 15%.
The differences in the frequency of CHOP on MCF-7 cell lines over time, and on circulating lymphocytes of patients with breast cancer, between the Propofol and Sevoflurane groups was analyzed by two-way repeated-measured ANOVA. For continuous variables, the distribution of the data was evaluated for normality using the Shapiro–Wilk test. The independent two-tailed t-test was used to compare means for continuous, normally distributed data between the Propofol and Sevoflurane groups. When the data were not normally distributed, the Mann–Whitney U test was used. Normally distributed continuous data are presented as the mean ± standard deviation; data that were not normally distributed are presented as the median (Q1, Q3). The chi-square test was used to compare the means of categorical variables between the two groups. For categorical variables, the number of patients (n) and proportion (%) were calculated. All calculations were performed using SPSS software (ver. 20.0; SPSS Inc., USA). A P value < 0.05 was taken to indicate statistical significance.
Eighty-four patients were eligible for the study, of whom 31 were excluded: 14 refused to participate, nine had abnormal preoperative laboratory results, four previously had cancer, one had a propofol allergy, and three underwent other types of concurrent surgery. Therefore, 53 patients were included in the final analysis (Fig. 1).
The patient demographics were similar between the Propofol and Sevoflurane groups (Table 1). The total amount of propofol was significantly higher in the Propofol than Sevoflurane group, and thiopental sodium was significantly higher in the Sevoflurane than Propofol group. The lowest and highest BIS values did not differ significantly between the two groups (Table 1). The amounts of medications, such as vasopressors, inotropics, opioids, and ketorolac, administered perioperatively were similar between the two groups (Table 2). The VAS and postoperative nausea and vomiting scores did not differ between the two groups during the study (Table 2).
The CHOP expression on MCF-7 cell lines did not differ between the Propofol and Sevoflurane groups (0.20% [0.14, 0.23%] vs. 0.13% [0.07, 0.21%], 0.22% [0.06, 0.24%] vs. 0.09% [0.05, 0.17%], and 0.14 ± 0.07 vs. 0.12 ± 0.07% preoperatively, 1 h postoperatively, and 24 h postoperatively, respectively; overall group difference (95% CI, 0.03 [−0.01, 0.07], P = 0.108)) (Fig. 2). The CHOP expression on MCF-7 cell lines in both groups decreased significantly over time (P = 0.027) (Fig. 2).
The CHOP expression on lymphocytes did not differ between the Propofol and Sevoflurane groups (0.16% [0.05, 0.41%] vs. 0.07% [0.03, 0.19%], 0.13% [0.07, 0.39%] vs. 0.04% [0.02, 0.13%], and 0.19% [0.08, 0.52%] vs. 0.05% [0.02, 0.38%] preoperatively, 1 h postoperatively, and 24 h postoperatively, respectively) (P = 0.485) (Fig. 3). In addition, the changes of CHOP expressions on lymphocytes in both groups showed no specific pattern over time (P = 0.836) (Fig. 3).
The ratios of neutrophils to leukocyte in the Propofol vs. Sevoflurane groups (55.3 ± 8.0% vs. 57.6 ± 6.7% and 57.5 ± 11.5% vs. 58.4 ± 11.0% preoperatively and 24 h postoperatively, respectively) and lymphocyte to leukocyte (36.2 ± 8.6% vs. 33.8 ± 6.1% and 34.1 ± 8.9% vs. 33.4 ± 9.6% preoperatively and 24 h postoperatively, respectively) did not differ significantly between the Propofol and Sevoflurane groups (P = 0.441 and P = 0.361, respectively) (Table 3). The ratios of neutrophils to lymphocytes (1.53 [1.16, 1.94] vs. 1.80 [1.48, 2.05], 1.63 [1.35, 2.20] vs. 1.62 [1.31, 2.31] preoperatively and 24 h postoperatively, respectively) also were not significantly different between the Propofol and Sevoflurane groups (overall difference [95% CI], −0.118 [−0.469, 0.232], P = 0.501) (Table 3).
In this study, propofol did not induce greater ER stress in cancer cells than sevoflurane during breast cancer surgery. The anesthetics reduced the ER stress of cancer cells over time, but did not influence the ER stress of lymphocytes. The ratios of neutrophils to lymphocytes among leukocytes did not differ between the two groups.
Numerous studies have shown that propofol is superior to volatile anesthetics in terms of reducing cancer progression [12–14]. The anti-inflammatory effects of propofol might help reduce cancer progression [15]. However, in other studies the type of anesthetic did not influence cancer cell progression [16,17]. In our study, the anti-inflammatory effect of propofol has not been reproduced in a clinical situation, although it has been demonstrated in animal studies. The cancer environment is complex and non-homogenous, such that the effects of anesthetics on cancer may not be dramatic. Several studies have evaluated the effects of various anesthetics on ER stress in different diseases. Propofol exerts an organ protective effect by modulating ER stress, although patterns of ER stress changes vary. Chen et al. [18] revealed that propofol enhanced ER stress and ultimately induced cell apoptosis of unnecessary muscle tissue to protect muscle. By contrast, Wang et al. [19] observed a neuroprotective effect of propofol through a reduction of ER stress and amelioration of neuronal cell apoptosis. Su et al. [20] showed an organ protective effect of propofol against ischemic reperfusion injury through a reduction of ER stress in normal cells. The up and down regulation of ER stress by propofol commonly induces ER stress related apoptosis of abnormal cells, which protect organs. Cui et al. [21] showed that propofol exerted anti-cancer effects by enhancing ER stress-related cancer cell apoptosis in a lung cancer model. However, in the present study, the ER stress of breast cancer cells was not higher under propofol-based anesthesia than volatile anesthetics-based anesthesia. In fact, independent of the anesthetic type, the ER stress of cancer cells decreased following anesthesia. We assume that this might be attributable to differences in the cell microenvironment among diseases and types of cancer. Li et al. [22] showed that regional anesthesia with bupivacaine reduced ER stress in colorectal cancer, but not in melanoma. In addition, our previous in vitro study showed that propofol reduced ER stress in normal tissues, but not cancer cells [23]. The cancer environment of breast cancer may differ from that of other malignancies in which changes in ER stress as a result of propofol have been reported.
This study showed that propofol and sevoflurane inhibit ER stress in cancer cell over time. The decrement in CHOP expression on cancer cells after anesthesia reflected reduced ER stress of cancer cells due to propofol- and sevoflurane-based anesthesia. Chevet et al. [24] found that, when the immune status was inadequate, ER stress contributed to cancer progression. Moreover, cancer-related hypoxia modulated ER stress, leading to cancer cell progression [25]. In most breast cancers, high levels of ER stress suppress cancer cell apoptosis [26,27]. However, we did not investigate the mechanism of decrement in ER stress after anesthesia. We assume that some cytokines and transcription factors can reduce ER stress after anesthesia [28].
There were several limitations to this study. First, the equipotent doses of propofol- and sevoflurane-based anesthesia were designed to achieve equivalent BIS value. However, whether these anesthetics have equivalent potency in terms of their effects on cancer cell ER stress is not clear. In clinical practice, the BIS provides a basis for clinical comparison between propofol and volatile anesthetics [29]. In several clinical studies, BIS values were maintained in the same range for propofol and volatile anesthetics, and equipotency was thus achieved [30-32]. However, we were unable to determine equivalent concentrations of these anesthetics in vitro. Nonetheless, we performed this study because the majority of anesthesiologists that use anesthetics ensure adequate BIS; anesthetics are always guided by the BIS or other kinds of processed electroencephalogram index during cancer surgery. We believe that referring to the BIS is the best option to ensure equipotency among diverse anesthetics. Second, we did not directly investigate the apoptosis of cancer cell lines. Moreover, we did not examine ER receptors such as inositol requiring enzyme-1α (IRE1α), double-strand RNA-dependent protein kinase (PERK), and activating transcription factor 6 (ATF6) [25], which sense ER stress. However, CHOP is a good marker of ER stress of cells [33]. Zheng et al. [34] showed that the CHOP level predicts disease free survival in breast cancer patients. Third, although the pro- and anti-apoptotic activities could differ among cancer cell lines, only one breast cancer cell line was cultured in this study [35]. Fourth, the mechanism underlying the decrement in ER stress seen in the cancer cell lines in this study after anesthesia was not identified. Possible mechanism related to ER stress of cancer cell after anesthesia should be evaluated in further studies. Finally, the effect of thiopental sodium on ER stress in the Sevoflurane group was not considered. Few studies have evaluated the effect of thiopental sodium on ER stress during cancer surgery [36]. However, a single dose of thiopental sodium during the early anesthesia period would have negligible effect on ER stress during cancer surgery.
In conclusion, propofol-based anesthesia did not induce greater ER stress of cancer cells than sevoflurane-based anesthesia in vitro setting. However, the ER stress of the cancer cells declined after anesthesia. These findings need to be validated in detailed studies explicitly investigating the effect of anesthesia on ER stress after cancer surgery.
Notes
Funding
This research was supported by the Basic Science Research Program through the National Research Foundation of Korea (NRF) funded by the Ministry of Science, Information & Communication Technology (Grant no: 2021R1A2C1003360).
Conflicts of Interest
Seong-Hyop Kim has been an editor for the Korean Journal of Anesthesiology since 2019. However, he was not involved in any process of review for this article, including peer reviewer selection, evaluation, or decision-making. There were no other potential conflicts of interest relevant to this article.
References
1. Biwer LA, Isakson BE. Endoplasmic reticulum-mediated signalling in cellular microdomains. Acta Physiol (Oxf). 2017; 219:162–75.
2. Iurlaro R, Muñoz-Pinedo C. Cell death induced by endoplasmic reticulum stress. FEBS J. 2016; 283:2640–52.


3. Oakes SA, Papa FR. The role of endoplasmic reticulum stress in human pathology. Annu Rev Pathol. 2015; 10:173–94.


4. Kim H, Bhattacharya A, Qi L. Endoplasmic reticulum quality control in cancer: friend or foe. Semin Cancer Biol. 2015; 33:25–33.


5. Cakmakkaya OS, Kolodzie K, Apfel CC, Pace NL. Anaesthetic techniques for risk of malignant tumour recurrence. Cochrane Database Syst Rev. 2014; (11):CD008877.


6. Wigmore TJ, Mohammed K, Jhanji S. Long-term survival for patients undergoing volatile versus IV anesthesia for cancer surgery: a retrospective analysis. Anesthesiology. 2016; 124:69–79.
7. Cruz FF, Rocco PR, Pelosi P. Anti-inflammatory properties of anesthetic agents. Crit Care. 2017; 21:67.


8. Alam A, Rampes S, Patel S, Hana Z, Ma D. Anesthetics or anesthetic techniques and cancer surgical outcomes: a possible link. Korean J Anesthesiol. 2021; 74:191–203.


9. Wang L, Tang W, Jiang T, Lu P, Li Y, Sun A, et al. Endoplasmic reticulum stress is involved in the neuroprotective effect of propofol. Neurochem Res. 2014; 39:1741–52.


10. Wang H, Dong Y, Zhang J, Xu Z, Wang G, Swain CA, et al. Isoflurane induces endoplasmic reticulum stress and caspase activation through ryanodine receptors. Br J Anaesth. 2014; 113:695–707.


11. Minto CF, Schnider TW, Egan TD, Youngs E, Lemmens HJ, Gambus PL, et al. Influence of age and gender on the pharmacokinetics and pharmacodynamics of remifentanil. I. Model development. Anesthesiology. 1997; 86:10–23.


12. Ecimovic P, McHugh B, Murray D, Doran P, Buggy DJ. Effects of sevoflurane on breast cancer cell function in vitro. Anticancer Res. 2013; 33:4255–60.
13. Huitink JM, Heimerikxs M, Nieuwland M, Loer SA, Brugman W, Velds A, et al. Volatile anesthetics modulate gene expression in breast and brain tumor cells. Anesth Analg. 2010; 111:1411–5.


14. Lee JH, Kang SH, Kim Y, Kim HA, Kim BS. Effects of propofol-based total intravenous anesthesia on recurrence and overall survival in patients after modified radical mastectomy: a retrospective study. Korean J Anesthesiol. 2016; 69:126–32.


15. Siddiqui RA, Zerouga M, Wu M, Castillo A, Harvey K, Zaloga GP, Stillwell W. Anticancer properties of propofol-docosahexaenoate and propofol-eicosapentaenoate on breast cancer cells. Breast Cancer Res . 2005; 7:R645–54.


16. Tylman M, Sarbinowski R, Bengtson JP, Kvarnstrom A, Bengtsson A. Inflammatory response in patients undergoing colorectal cancer surgery: the effect of two different anesthetic techniques. Minerva Anestesiol. 2011; 77:275–82.
17. Oh CS, Lee J, Yoon TG, Seo EH, Park HJ, Piao L, et al. Effect of equipotent doses of propofol versus sevoflurane anesthesia on regulatory t cells after breast cancer surgery. Anesthesiology. 2018; 129:921–31.
18. Chen X, Li LY, Jiang JL, Li K, Su ZB, Zhang FQ, et al. Propofol elicits autophagy via endoplasmic reticulum stress and calcium exchange in C2C12 myoblast cell line. PloS One. 2018; 13:e0197934.


19. Wang B, Ge S, Xiong W, Xue Z. Effects of resveratrol pretreatment on endoplasmic reticulum stress and cognitive function after surgery in aged mice. BMC Anesthesiol. 2018; 18:141.


20. Su M, Ren S, Zhong W, Han X. Impact of propofol on renal ischemia/reperfusion endoplasmic reticulum stress. Acta Cir Bras. 2017; 32:533–9.


21. Cui WY, Liu Y, Zhu YQ, Song T, Wang QS. Propofol induces endoplasmic reticulum (ER) stress and apoptosis in lung cancer cell H460. Tumour Biol. 2014; 35:5213–7.


22. Li T, Chen L, Zhao H, Wu L, Masters J, Han C, et al. Both Bupivacaine and Levobupivacaine inhibit colon cancer cell growth but not melanoma cells in vitro. J Anesth. 2019; 33:17–25.


23. Seo EH, Piao L, Park HJ, Lee JY, Sa M, Oh CS, et al. Impact of general anaesthesia on endoplasmic reticulum stress: propofol vs. isoflurane. Int J Med Sci. 2019; 16:1287–94.
24. Chevet E, Hetz C, Samali A. Endoplasmic reticulum stress-activated cell reprogramming in oncogenesis. Cancer Discov. 2015; 5:586–97.


25. Madden E, Logue SE, Healy SJ, Manie S, Samali A. The role of the unfolded protein response in cancer progression: From oncogenesis to chemoresistance. Biol Cell. 2019; 111:1–17.


26. Déry MA, Jodoin J, Ursini-Siegel J, Aleynikova O, Ferrario C, Hassan S, et al. Endoplasmic reticulum stress induces PRNP prion protein gene expression in breast cancer. Breast Cancer Res. 2013; 15:R22.
27. Lee E, Nichols P, Spicer D, Groshen S, Yu MC, Lee AS. GRP78 as a novel predictor of responsiveness to chemotherapy in breast cancer. Cancer Res. 2006; 66:7849–53.


28. Ku HC, Cheng CF. Master regulator activating transcription factor 3 (ATF3) in metabolic homeostasis and cancer. Front Endocrinol (Lausanne). 2020; 11:556.
29. Glass PS, Bloom M, Kearse L, Rosow C, Sebel P, Manberg P. Bispectral analysis measures sedation and memory effects of propofol, midazolam, isoflurane, and alfentanil in healthy volunteers. Anesthesiology. 1997; 86:836–47.


30. Law-Koune JD, Raynaud C, Liu N, Dubois C, Romano M, Fischler M. Sevoflurane-remifentanil versus propofol-remifentanil anesthesia at a similar bispectral level for off-pump coronary artery surgery: no evidence of reduced myocardial ischemia. J Cardiothorac Vasc Anesth. 2006; 20:484–92.


31. Oh CS, Park HJ, Piao L, Sohn KM, Koh SE, Hwang DY, et al. Expression profiles of immune cells after propofol or sevoflurane anesthesia for colorectal cancer surgery: a prospective double-blind randomized trial. Anesthesiology. 2022; 136:448–58.


32. Kadoi Y, Kawauchi C, Saito S, Takahashi K. The comparative effects of equipotent Bispectral Index dosages of propofol and sevoflurane on cerebrovascular carbon dioxide reactivity in elderly patients. J Clin Anesth. 2009; 21:173–7.
33. Tang C, Hu Y, Gao J, Jiang J, Shi S, Wang J, et al. Dexmedetomidine pretreatment attenuates myocardial ischemia reperfusion induced acute kidney injury and endoplasmic reticulum stress in human and rat. Life Sci. 2020; 257:118004.


34. Zheng YZ, Cao ZG, Hu X, Shao ZM. The endoplasmic reticulum stress markers GRP78 and CHOP predict disease-free survival and responsiveness to chemotherapy in breast cancer. Breast Cancer Res Treat. 2014; 145:349–58.


35. Comşa Ş, Cîmpean AM, Raica M. The story of MCF-7 breast cancer cell line: 40 years of experience in research. Anticancer Res. 2015; 35:3147–54.
Fig. 2.
CHOP expression on MCF-7 cell lines in both groups. Values in this figure are presented as mean (95% CI). The CHOP expression on MCF-7 cell lines in the Propofol and Sevoflurane groups were 0.18% (0.04%) vs. 0.16% (0.05%), 0.17% (0.04%) vs. 0.11% (0.03%), and 0.14% (0.03%) vs. 0.12% (0.03%) at Preop, Post 1 h, and Post 24 h, respectively. *P = 0.108 (group difference across all measured time points). †P = 0.027 (comparison with preoperative levels). CHOP: C/EBP homologous protein, MCF-7 cell line: Michigan Cancer Foundation-7 human breast cancer cell line, Preop: before inducing anesthesia, Post 1 h: on arrival at the post-anesthesia care unit, Post 24 h: 24 h postoperatively.
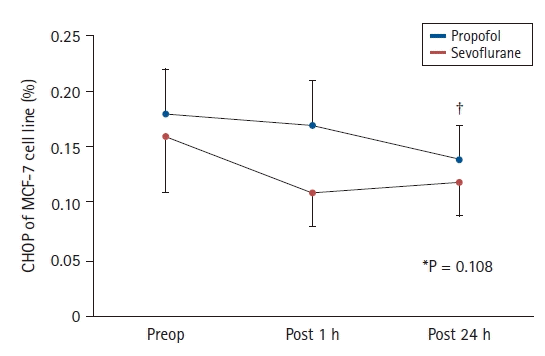
Fig. 3.
CHOP expression on lymphocytes in both groups. Values in this figure are presented as mean (95% CI). The CHOP expression on lymphocytes in the Propofol and Sevoflurane groups were 0.3% (0.14%) vs. 0.28% (0.23%), 0.29% (0.15%) vs. 0.22% (0.20%), and 0.34% (0.15%) vs. 0.23% (0.15%) at Preop, Post 1 h, and Post 24 h, respectively. *P = 0.485 (group differences across all measured time points). CHOP: C/EBP homologous protein, Preop: before inducing anesthesia induction, Post 1 h: on arrival at the post-anesthesia care unit, Post 24 h: 24 h postoperatively.
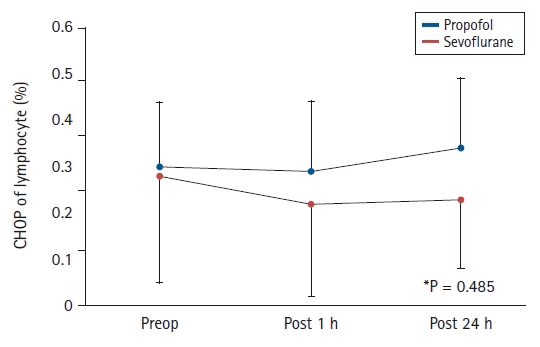
Table 1.
Demographic Characteristics of the Propofol- and Sevoflurane-based Anesthesia during Breast Cancer Surgery
Table 2.
Amount of Medications Administered and Postoperative Pain and Nausea/vomiting
Table 3.
The Changes in Neutrophil and Lymphocyte during Breast Surgery