Abstract
Background
The neocortex, including the medial prefrontal cortex (mPFC), contains many neurons expressing nitric oxide synthase (NOS). In addition, increasing evidence shows that the nitric oxide (NO) and opioid systems interact in the brain. However, there have been no studies on the interaction of the opioid and NO systems in the mPFC. The objective of this study was to investigate the effects of administrating L-arginine (L-Arg, a precursor of NO) and N(gamma)-nitro-L-arginine methyl ester (L-NAME, an inhibitor of NOS) into the mPFC for neuropathic pain in rats. Also, we used selective opioid receptor antagonists to clarify the possible participation of the opioid mechanism.
Methods
Complete transection of the peroneal and tibial branches of the sciatic nerve was applied to induce neuropathic pain, and seven days later, the mPFC was cannulated bilaterally. The paw withdrawal threshold fifty percent (50% PWT) was recorded on the 14th day.
Results
Microinjection of L-Arg (2.87, 11.5 and 45.92 nmol per 0.25 µL) increased 50% PWT. L-NAME (17.15 nmol per 0.25 µL) and naloxonazine (an antagonist of mu opioid receptors, 1.54 nmol per 0.25 µL) inhibited anti-allodynia induced by L-Arg (45.92 nmol per 0.25 µL). Naltrindole (a delta opioid receptor antagonist, 2.45 nmol per 0.25 µL) and nor-binaltorphimine (a kappa opioid receptor antagonist, 1.36 nmol per 0.25 µL) were unable to prevent L-Arg (45.92 nmol per 0.25 µL)-induced antiallodynia.
Many complex functions of the brain are regulated by nitric oxide (NO), a gaseous neurotransmitter. L-arginine (L-Arg) is a natural precursor for the synthesis of NO. In mammalian cells, the NO synthase (NOS) family of enzymes catalyzes conversion of L-Arg to NO [1]. Interestingly, several studies have shown that the NO system participates in the acute and chronic states of pain either in the peripheral or the central nervous systems [1,2]. On the other hand, functionally, interaction of NO and opioid systems, and NO modulation of opioid antinociceptive tolerance were documented in several studies. For example, when administered along with morphine, N(gamma)-nitro-L-arginine methyl ester (L-NAME) prevents tolerance to morphine and also counteracts some signs of morphine dependence in mice [3]. Furthermore, a chronic nutritional supplement with the NO precursor, L-Arg, restored the potency and efficacy of morphine in rats with advanced diabetes [4].
The medial, lateral, and ventral (orbitofrontal) parts are the main sub-regions of the prefrontal cortex (PFC) [5]. The medial part of the PFC (mPFC) participates in many brain-related tasks such as memory and learning, planning and decision-making, emotional processing, and anxiety [5,6]. In addition, the role of PFC and notably the mPFC in modulation of acute and chronic pain was reported [7,8]. The mPFC receives ascending, nociceptive inputs and also sends projections to control the descending inhibitory pain pathways [9]. In this regard, it has been found that cannabinoid and mu-opioid receptors in the mPFC and periaqueductal gray (PAG) cooperated in diclofenac-induced antinociception in the mPFC [10]. In addition, in persistent back pain patients, functional connectivity of the mPFC was changed, and these alterations were linked to duration and severity of pain, indicating that the mPFC may be a target in pain processing [11].
The International Association for the Study of Pain describes neuropathic pain as pain caused by damage or disease of the somatosensory nervous system [12]. Diabetic neuropathy, radiculopathies, trigeminal neuralgia, postherpetic neuralgia, and post-amputation pain are included in neuropathic pain. Allodynia and hyperalgesia are two particularly prominent symptoms in different types of neuropathic pain [13]. Several animal models such as chronic constriction injury and spared nerve injury (SNI) have been developed for the study of this type of pain [14].
NO signaling in the mPFC is investigated in control of several elaborated central functions such as anxiety, depression, fear formation, and some cognitive dysfunctions [15,16]. Furthermore, there is little evidence suggesting a role for the NO system of this area in pain regulation. For example, the nNOS-expressing neurons of the ventromedial PFC receive chronic inflammatory pain signals from the paraventricular thalamic nucleus and transform them into anxiety behaviors [17]. However, the involvement of the NO system of the mPFC in pain modulation has not been clearly understood.
Many neurons in the neocortex, including those in the mPFC, express the NOS [17]. In addition, the NO is a key neurotransmitter in the pain process that has the ability to reduce inflammatory pain as well as neuropathic pain [2]. Therefore, this study was carried out to evaluate the effects of separate and combined infusion of L-NAME (an NOS inhibitor) and L-Arg (a precursor of NO) into the mPFC on neuropathic pain induced by SNI in rats. In addition, the contribution of opioid receptors was assessed using naloxonazine, naltrindole, and nor-binaltorphimine (selective mu, delta, and kappa opioid receptor antagonists, respectively). The effects of these agents on locomotor activity were also assessed using an animal electronic motor apparatus.
Adult albino rats of the Wistar strain (12 to 14 months-old male rats, weighing 265 ± 10 g) were supplied by the animal house of the Laboratory of Physiology. The animals were placed in cages made from polyethylene, without any restriction to water and food, and under a light-dark cycle (12/12 hours). The room temperature was maintained between 21 and 23 degrees centigrade. We conducted our experiments between 12:30 p.m. and 4:30 p.m. All animal care procedures were carried out according to standard animal experimentation protocol (IR-UU-AEC-149/PD/3).
L-Arg (PubChem CID: 6322), L-NAME hydrochloride (PubChem CID: 135193), nor-binaltorphimine hydrochloride (PubChem CID: 5480230), naltrindole hydrochloride (PubChem CID: 24840086), and naloxonazine dihydrochloride (PubChem CID: 11957656) (Sigma-Aldrich, St. Louis, MO) were used in this study. Sterile normal saline (sodium chloride 0.9% solution, PubChem CID: 5234) was used for dilution of drugs.
Based on previous published studies [18,19], the experimental schedule of our study is shown in Fig. 1. According to study protocol, sham and SNI surgeries were conducted on the first day. Seven days later, the rats received bilateral cannulation of the mPFC for normal saline and drugs infusion. On the 14th day following SNI surgery, mechanical allodynia was assessed before and after normal saline and drug solutions delivery. Six days later, the locomotor activity of the rats was evaluated. To verify microinjection locations, the rats were euthanized, and then their brains were taken out when the experiments were over.
Animals were anesthetized with a ketamine and xylazine cocktail (intraperitoneal [i.p.], 80 mg kg-1 and 8 mg kg-1, respectively [Alphasan, Woerden, Netherlands]), and the thigh was incised along the back. By passing through the biceps femoris muscle, three distal sciatic nerve branches were visible. The SNI was performed by the ligating and axotomy of the tibial and common peroneal nerves, leaving the sural nerve intact, as previously described [18,19]. In sham surgery rats, the sciatic nerve branches were exposed but not injured. The rats underwent recovery before intra-mPFC cannula implantation surgery.
On the seventh day, rats were anesthetized with a ketamine and xylazine cocktail (i.p., 80 mg kg-1 and 8 mg kg-1, respectively) and the rats were then placed in a stereotaxic apparatus (Stoelting, Wood Dale, IL). The mPFC were implanted bilaterally (right and left sides) with 24-gauge, 12-mm-length guide cannulas in accordance with information derived from the atlas of the rat brain [20] measured relative to the bregma: 3.2 mm anterior, ± 1.6 mm lateral, and 3.3 mm ventral, with a 15 degree angle in the lateral plan. Bone screws and dental acrylic cement were used to attach the cannula to the skull. The rats were given seven days to recover before behavioral testing.
All drug solutions were infused into the mPFC at a slow speed of 45 seconds at a total volume of 0.25 μL via a 30 gauge needle, which was 1 mm longer than guide cannulas and attached to a 1 μL Hamilton syringe. In addition, backflow of the drug was prevented by holding the needle in place for an additional 45 seconds.
On day 14 after SNI induction, mechanical allodynia was applied to measure the sensory component of neuropathic pain. The up-down Von Frey method was performed to determine a 50% paw withdrawal response as described previously [19,23,24]. Rats were placed in a plexiglass chamber fixed above a wire mesh floor and allowed to adapt to the apparatus for 30 minutes prior to observation. Nociceptive testing performance was based on a previous experiment [19]. In brief, we chose eleven von Frey filaments (von Frey numbers: 3-13; IITC-Life Science, Woodland Hills, CA). In the beginning, number 8 (an intermediate filament, equivalent to 2.5 g) was applied and the number of the filament was increased or decreased depending on the response of the animal. In a five-second time interval, filaments were pressed repeatedly from below and against the lateral area (the sural nerve innervation region) of the hind paw. If no response occurred, the next stiffer fiber was used, and if a response occurred, a less stiff fiber was applied. After application of a stiffer fiber, when there was no response, a less stiff fiber was used and vice versa. Chaplan’s formula [24] allowed negative and positive responses to be converted to a threshold of 50%.
On the 20th day, we used an electronic system (Borj Sanat, Tehran, Iran), including a Plexiglas box (40 × 40 × 40 cm), to evaluate the locomotor behavior of the animals. A moving animal caused photo beam breaks in the box, and the number of broken beams in a 5-minute session was shown on the monitor of the apparatus.
At the end of the experiments, the implantation of the cannula in the mPFC was verified. Rats were deeply anesthetized with a mixture of xylazine and ketamine and then were euthanized. Brains were removed, their dorsal surfaces photographed and then stored in formalin solution. Seven days later, thin transverse sections (50–100 μm) were provided. Sections were viewed under a loupe to determine the location of the cannulas aimed for the site of microinjection (mPFC) according to the atlas of rat brain [20]. Our analysis did not include the data from the rats whose cannulas were not positioned correctly.
Software GraphPad Prism, version 5 used for statistical analysis (GraphPad Prism Software, San Diego, CA) and a P value less than 0.05 was considered significant. For data obtained from 20-min time points (paw withdrawal threshold fifty percent [50% PWT]), we used a repeated measures two-way analysis of variance (ANOVA) followed by the Bonferroni post hoc test. In addition, the area under the curve (AUC) was calculated by the trapezoid area method and one-way ANOVA and Tukey post hoc tests were used to assess data obtained from the AUC. A dose-response curve was also prepared by GraphPad Prism software. Values represent the mean with SEM.
The 50% PWT declined to 3.013 ± 0.33 g (n = 6) two weeks after rat SNI surgery in comparison with 12.05 ± 0.32 g (n = 6) in rats with no SNI (sham surgery animals). In addition, normal saline infusion into the mPFC had no significant effect on SNI-induced allodynia and the 50% PWT was preserved at the pre-microinjection control range (2.84 ± 0.09) at all times after intra-mPFC infusion of normal saline.
Fig. 3A showed significant differences between time (F4,150 = 70.51, P < 0.001), treatments (F5,150 = 579.2, P < 0.001) and interactions (F20,150 = 19.74, P < 0.001). Further analysis revealed that L-Arg (0.72 nmol) did not alter pain response, whereas at a dose of 2.87 nmol increased mean 50% PWT at 25, 45, and 65 minutes time points, and at doses of 11.5 and 45.92 nmol at all-time points it increased 50% PWT after microinjection (Fig. 3A). In addition, the related AUC confirmed the above-mentioned drug treatment effects (F5,35 = 507.8 , P < 0.001, Fig. 3B). As shown in Fig. 3C, the percentage of the response in which L-Arg caused anti-allodynia increased dose dependently.
Fig. 4A showed significant differences between time (F4,150 = 47.81, P < 0.001), treatments (F5,150 = 632.26, P < 0.001), and interactions (F20,150 = 23.32, P < 0.001) at all-time points. Further statistical analysis showed that microinjection of L-NAME at doses of 4.25 and 17.15 nmol into the mPFC did not affect mean 50% PWT compared to the normal saline-treated group (Fig. 4A). However, prior L-NAME (17.15 nmol) microinjection prevented the anti-allodynic effect of L-Arg (45.92 nmol) at all-time points (Fig. 4A). Moreover, the above-mentioned drug treatment effects were confirmed by the related AUC (F5,35 = 1,428, P < 0.001, Fig. 4B).
Fig. 5A showed significant differences between time (F4,125 = 29.14, P < 0.001), treatments (F4,125 = 639.38, P < 0.001) and interactions (F16,125 = 22.00, P < 0.001) at all-time points. Additional data analysis revealed that mechanical allodynia induced by SNI was not changed by the mPFC infusion of 1.54 nmol of naloxonazine with respect to the normal saline-treated group. On the other hand, pre-treatment with naloxonazine (1.54 nmol) significantly (P < 0.001) prevented the anti-allodynic effect of L-Arg (45.92 nmol) at all-time points. As shown in Fig. 5B and 5C, microinjections of nor-binaltorphimine (1.36 nmol) and naltrindole (2.41 nmol) into the mPFC did not change the mechanical allodynia induced by SNI in comparison with the normal saline-treated group. In addition, pre-treatment of nor-binaltorphimine (1.36 nmol) and naltrindole (2.41 nmol) into mPFC did not alter the anti-allodynic effect of L-Arg (45.92 nmol). The above-mentioned drug treatment effects were confirmed by the related AUC (F8,35 = 684.1, P < 0.001, Fig. 5D).
The number of infrared beam breaks in the intra-mPFC normal saline-treated group was 83.33 ± 3.68. Neither the separate nor combined treatments affected locomotor activity (Fig. 6).
Based on our results, microinjection of L-Arg into the mPFC suppressed mechanical allodynia. Microinjection of L-NAME itself did not affect mechanical allodynia; however, L-NAME pre-treatment inhibited L-Arg-induced anti-allodynic effect. These findings suggest that at the mPFC level, L-Arg-induced antinociception is probably related to activation of the NO system.
In pain processing, the PFC plays an essential role due to its extensive connections with different brain areas such as the thalamus, hippocampal formation, PAG and cerebral neocortex [8,25]. In addition, the antinociceptive properties of several neurotransmitters in this area have been well documented. In this regard, SNI-induced neuropathy leads to increased expression of inflammatory markers, including inducible NOS in M1 microglia in the PFC [26].
NO exerts its effect by activating a series of intracellular pathways. A critical pathway is the soluble guanylyl cyclase/cyclic guanosine mono-phosphate/protein kinase G system [27]. The enzyme NOS catalyzes NO production from L-Arg. The NO signaling pathway has been shown to play an essential role in pain processing and participates in the drug-induced antinociception [1,27–29]. In this regard, central and systemic inhibition of synthesis by L-NAME reverses the antinociceptive effects of different drugs. Centrally administered L-NAME 15 minutes before an intracerebroventricular (i.c.v.) microinjection of thymoquinone inhibited the antinociceptive effects of thymoquinone in both phases of the formalin test [27]. Similar results also have been obtained for systemic administration of L-NAME prior to dexketoprofen in both the murine tail-flick and writing tests [29]. On the other hand, Fakhri et al. [30] reported that systemic administrations of L-Arg, S-nitroso-N-acetylpenicillamine (SNAP, an NO donor), and sildenafil (a phosphodiesterase inhibitor) increased and L-NAME inhibited the antinociceptive effect of melatonin, suggesting that melatonin’s antinociceptive effects in formalin pain probably originate from the NO signaling pathway. Similarly, the antinociceptive effect of astaxanthin was reversed by L-NAME and glibenclamide and this suggests that the NO signaling pathway participates in the antinociceptive effects of astaxnathin [31].
In the central and peripheral nervous systems, studies have documented that neuronal and non-neuronal origin NO play complex roles in the modulation of pain perception [1]. The mechanisms involved in this effect of NO have not been fully elucidated. Evidence for the central antinociceptive effects of NO suggests a possible role for NO in the descending system of pain and its interaction with noradrenergic and cholinergic modulation in this pathway [32–34]. Researchers reported that an i.c.v. injection of L-Arg had an antinociceptive effect on carrageenan-induced hyperalgesia [35]. However, the importance of the NO system of the PFC in neuropathic pain has not been investigated yet. In our study, inhibition of the anti-allodynic effect of L-Arg by L-NAME suggests that L-Arg exerts its anti-allodynic effect by activating the NO system. However, there are some reports that L-NAME, in systemic and spinal administrations, produced antinociception in animal models of pain [36,37]. This inconsistency may relate to doses used in their study and non-brain mechanisms of antinociception.
In this study, microinjection of naloxonazine, naltrindol, and nor-binaltorphimine alone into the mPFC did not alter SNI-induced allodynia; however, prior microinjection of naloxonazine into the mPFC prevented the L-Arg-induced anti-allodynic effect. It has been shown that opioids in all animal species are derived from the three major propeptides, proenkephalin A and B, and proopiomelanocortin, and bind to the three main types of opioid receptors [38]. Central mu, kappa, and delta receptors are responsible for the antinociceptive effects induced by opioids [39]. To identify which type of opioid receptor might be involved in L-Arg-induced antinociception, we used selective opioid receptor antagonists (naloxonazine, naltrindol, and nor-binaltorphimene). Multiple data sources suggested interaction among NO and opioids. NO has been reported to mediate the antinociceptive effect of compounds, such as opioids and anti-inflammatory agents. For example, it was reported that naloxone could inhibit the antinociceptive effect of subcutaneously administrated L-Arg on carrageenan-induced hyperalgesia [35]. Also, an i.c.v. injection of L-Arg and the NO donor 3-morpholinosydnoimine had antinociceptive effects in laboratory mice that were blocked by naloxone and nor-binaltorphimine [40]. Moreover, it was found that the antinociceptive effect of morphine administered into the ventrolateral periaqueductal grey was reduced by prior L-NAME and MK801 microinjections into the rostral ventromedial medulla, indicating that supra-spinal NO production regulates the transmission of opioid pain inhibitory signals from the PAG [41]. At the hippocampal level, it was reported that the nociception caused by naloxone injection was inhibited by prior microinjection of L-Arg, and that L-NAME prevented this inhibition, indicating possible interaction between the effects of NO and the opioid systems at the brain regions [42].
In peripheral inflammation and neuropathic pain, the NO system has been reported to be involved in the antinociceptive effects and expression of opioid mu receptors [43,44]. For example, while sciatic nerve injury did not change mu opioid receptor mRNA and protein expression in the dorsal root ganglia in NOS knockout mice when comparing sciatic nerve-injured with sham-operated animals, it significantly decreases their levels in wild type mice [44]. In addition, mu opioid receptor gene expression was significantly down-regulated in human mononuclear cells (isolated from whole blood) treated with SNAP (a NO donor) [45]. Furthermore, an analgesic neuropeptide in the brain, kyotorphin, can be formed from L-Arg, which increases the release of MET-enkephalin in the brain [35]. The combined treatment of L-Arg and morphine may accelerate morphine tolerance, and chronic administration of L-Arg induces tolerance in naive mice [46]. Moreover, studies showed that NO appears to be a key modulator of morphine tolerance and elevations of NO levels in the brain in response to administration of L-Arg up-regulated the mu opioid receptors [47]. The study of Huang et al. [48] revealed that NO also modulates mu opioid receptor function in vitro. However, no study has been reported in the mPFC on the interaction of opioids and the NO systems.
The PFC, along with the anterior cingulate, the somatosensory I and II areas, and the insular cortices are among the most important areas of the brain activated during pain processing [8,10]. In addition, studies showed an association between the mPFC and neuropathic pain [7,49]. Based on the results of this study, the NO system in the mPFC can play a role in reducing neuropathic pain. One of the possible mechanisms involved in modulating neuropathic pain by this system is probably the activation of mu opioid receptors. Further studies are needed to understand other possible mechanisms. If we know more about NO and opioid interaction, we can formulate strategies for maximizing the analgesic effects of opioids while minimizing their related side effects.
Notes
REFERENCES
1. Cury Y, Picolo G, Gutierrez VP, Ferreira SH. 2011; Pain and analgesia: the dual effect of nitric oxide in the nociceptive system. Nitric Oxide. 25:243–54. DOI: 10.1016/j.niox.2011.06.004. PMID: 21723953. PMID: https://www.scopus.com/inward/record.uri?partnerID=HzOxMe3b&scp=80053919221&origin=inward.


2. Spiller F, Oliveira Formiga R, Fernandes da Silva Coimbra J, Alves-Filho JC, Cunha TM, Cunha FQ. 2019; Targeting nitric oxide as a key modulator of sepsis, arthritis and pain. Nitric Oxide. 89:32–40. DOI: 10.1016/j.niox.2019.04.011. PMID: 31051258. PMID: https://www.scopus.com/inward/record.uri?partnerID=HzOxMe3b&scp=85065091186&origin=inward.


3. Toda N, Kishioka S, Hatano Y, Toda H. 2009; Modulation of opioid actions by nitric oxide signaling. Anesthesiology. 110:166–81. DOI: 10.1097/ALN.0b013e31819146a9. PMID: 19104184. PMID: https://www.scopus.com/inward/record.uri?partnerID=HzOxMe3b&scp=58749107193&origin=inward.


4. Lotfipour S, Smith MT. 2018; Morphine hyposensitivity in streptozotocin-diabetic rats: reversal by dietary l-arginine treatment. Clin Exp Pharmacol Physiol. 45:42–9. DOI: 10.1111/1440-1681.12855. PMID: 28925511. PMID: https://www.scopus.com/inward/record.uri?partnerID=HzOxMe3b&scp=85032259148&origin=inward.


5. Salighedar R, Erfanparast A, Tamaddonfard E, Soltanalinejad F. 2019; Medial prefrontal cortex oxytocin-opioid receptors interaction in spatial memory processing in rats. Physiol Behav. 209:112599. DOI: 10.1016/j.physbeh.2019.112599. PMID: 31276680. PMID: https://www.scopus.com/inward/record.uri?partnerID=HzOxMe3b&scp=85068563144&origin=inward.


6. Xu P, Chen A, Li Y, Xing X, Lu H. 2019; Medial prefrontal cortex in neurological diseases. Physiol Genomics. 51:432–42. DOI: 10.1152/physiolgenomics.00006.2019. PMID: 31373533. PMCID: PMC6766703. PMID: https://www.scopus.com/inward/record.uri?partnerID=HzOxMe3b&scp=85071788325&origin=inward.


7. Qian Y, Wang Z, Zhou S, Zhao W, Yin C, Cao J, et al. 2020; MKP1 in the medial prefrontal cortex modulates chronic neuropathic pain via regulation of p38 and JNK1/2. Int J Neurosci. 130:643–52. DOI: 10.1080/00207454.2019.1667785. PMID: 31518515. PMID: https://www.scopus.com/inward/record.uri?partnerID=HzOxMe3b&scp=85084310971&origin=inward.


8. Ong WY, Stohler CS, Herr DR. 2019; Role of the prefrontal cortex in pain processing. Mol Neurobiol. 56:1137–66. DOI: 10.1007/s12035-018-1130-9. PMID: 29876878. PMCID: PMC6400876. PMID: https://www.scopus.com/inward/record.uri?partnerID=HzOxMe3b&scp=85048062121&origin=inward.


9. Kummer KK, Mitrić M, Kalpachidou T, Kress M. 2020; The medial prefrontal cortex as a central hub for mental comorbidities associated with chronic pain. Int J Mol Sci. 21:3440. DOI: 10.3390/ijms21103440. PMID: 32414089. PMCID: PMC7279227. PMID: 62fc386c77484ecfaecb885d534a537c. PMID: https://www.scopus.com/inward/record.uri?partnerID=HzOxMe3b&scp=85084787528&origin=inward.


10. Tamaddonfard E, Erfanparast A, Salighedar R, Tamaddonfard S. 2020; Medial prefrontal cortex diclofenac-induced antinociception is mediated through GPR55, cannabinoid CB1, and mu-opioid receptors of this area and periaqueductal gray. Naunyn Schmiedebergs Arch Pharmacol. 393:371–9. DOI: 10.1007/s00210-019-01735-x. PMID: 31641818. PMID: https://www.scopus.com/inward/record.uri?partnerID=HzOxMe3b&scp=85074282153&origin=inward.


11. Tu Y, Jung M, Gollub RL, Napadow V, Gerber J, Ortiz A, et al. 2019; Abnormal medial prefrontal cortex functional connectivity and its association with clinical symptoms in chronic low back pain. Pain. 160:1308–18. Erratum in: Pain 2020; 161: 230-1. DOI: 10.1097/j.pain.0000000000001507. PMID: 31107712. PMCID: PMC6530583. PMID: https://www.scopus.com/inward/record.uri?partnerID=HzOxMe3b&scp=85063572752&origin=inward.


12. Scholz J, Finnerup NB, Attal N, Aziz Q, Baron R, Bennett MI, et al. 2019; The IASP classification of chronic pain for ICD-11: chronic neuropathic pain. Pain. 160:53–9. DOI: 10.1097/j.pain.0000000000001365. PMID: 30586071. PMCID: PMC6310153. PMID: https://www.scopus.com/inward/record.uri?partnerID=HzOxMe3b&scp=85056353013&origin=inward.


13. Jensen TS, Finnerup NB. 2014; Allodynia and hyperalgesia in neuropathic pain: clinical manifestations and mechanisms. Lancet Neurol. 13:924–35. DOI: 10.1016/S1474-4422(14)70102-4. PMID: 25142459. PMID: https://www.scopus.com/inward/record.uri?partnerID=HzOxMe3b&scp=84906076303&origin=inward.


14. Challa SR. 2015; Surgical animal models of neuropathic pain: pros and cons. Int J Neurosci. 125:170–4. DOI: 10.3109/00207454.2014.922559. PMID: 24831263. PMID: https://www.scopus.com/inward/record.uri?partnerID=HzOxMe3b&scp=84924227883&origin=inward.


15. Vila-Verde C, Marinho AL, Lisboa SF, Guimarães FS. 2016; Nitric oxide in the prelimbic medial prefrontal cortex is involved in the anxiogenic-like effect induced by acute restraint stress in rats. Neuroscience. 320:30–42. DOI: 10.1016/j.neuroscience.2016.01.040. PMID: 26812037. PMID: https://www.scopus.com/inward/record.uri?partnerID=HzOxMe3b&scp=84957922299&origin=inward.


16. Liu W, Li Q, Ye B, Cao H, Shen F, Xu Z, et al. 2020; Repeated nitrous oxide exposure exerts antidepressant-like effects through neuronal nitric oxide synthase activation in the medial prefrontal cortex. Front Psychiatry. 11:837. DOI: 10.3389/fpsyt.2020.00837. PMID: 33088274. PMCID: PMC7495238. PMID: 33bc10b3f8184aaf97f3b1e27172d0a2. PMID: https://www.scopus.com/inward/record.uri?partnerID=HzOxMe3b&scp=85091074996&origin=inward.


17. Liang HY, Chen ZJ, Xiao H, Lin YH, Hu YY, Chang L, et al. 2020; nNOS-expressing neurons in the vmPFC transform pPVT-derived chronic pain signals into anxiety behaviors. Nat Commun. 11:2501. DOI: 10.1038/s41467-020-16198-5. PMID: 32427844. PMCID: PMC7237711. PMID: 8f56e6d3aab84052a130814cd8153048. PMID: https://www.scopus.com/inward/record.uri?partnerID=HzOxMe3b&scp=85084963733&origin=inward.


18. Salimi S, Tamaddonfard E. 2019; Microinjection of histamine and its H3 receptor agonist and antagonist into the agranular insular cortex influence sensory and affective components of neuropathic pain in rats. Eur J Pharmacol. 857:172450. DOI: 10.1016/j.ejphar.2019.172450. PMID: 31202805. PMID: https://www.scopus.com/inward/record.uri?partnerID=HzOxMe3b&scp=85067298164&origin=inward.


19. Taati M, Tamaddonfard E. 2018; Ventrolateral orbital cortex oxytocin attenuates neuropathic pain through periaqueductal gray opioid receptor. Pharmacol Rep. 70:577–83. DOI: 10.1016/j.pharep.2017.12.010. PMID: 29679881. PMID: https://www.scopus.com/inward/record.uri?partnerID=HzOxMe3b&scp=85045624471&origin=inward.


20. Paxinos G, Watson C. 2007. The rat brain in stereotaxic coordinates. 6th ed. Elsevier;New York: DOI: 10.1016/j.pharep.2017.12.010. PMID: https://www.scopus.com/inward/record.uri?partnerID=HzOxMe3b&scp=85045624471&origin=inward.
21. Erfanparast A, Tamaddonfard E, Seyedin S. 2018; Involvement of central opiate receptors in modulation of centrally administered oxytocin-induced antinociception. Iran J Basic Med Sci. 21:1275–80. DOI: 10.22038/ijbms.2018.26302.6449. PMID: 30627372. PMCID: PMC6312677. PMID: https://www.scopus.com/inward/record.uri?partnerID=HzOxMe3b&scp=85055658537&origin=inward.
22. Karimi S, Karami M, Sahraei H, Rahimpour M. 2011; Reversal effect of intra-central amygdala microinjection of L-arginine on place aversion induced by naloxone in morphine conditioned rats. Iran Biomed J. 15:92–9. PMID: 21987115. PMCID: PMC3639744. PMID: https://www.scopus.com/inward/record.uri?partnerID=HzOxMe3b&scp=82555188426&origin=inward.
23. Dixon WJ. 1980; Efficient analysis of experimental observations. Annu Rev Pharmacol Toxicol. 20:441–62. DOI: 10.1146/annurev.pa.20.040180.002301. PMID: 7387124. PMID: https://www.scopus.com/inward/record.uri?partnerID=HzOxMe3b&scp=0018867072&origin=inward.


24. Chaplan SR, Bach FW, Pogrel JW, Chung JM, Yaksh TL. 1994; Quantitative assessment of tactile allodynia in the rat paw. J Neurosci Methods. 53:55–63. DOI: 10.1016/0165-0270(94)90144-9. PMID: 7990513. PMID: https://www.scopus.com/inward/record.uri?partnerID=HzOxMe3b&scp=0027933374&origin=inward.


25. Bastuji H, Frot M, Perchet C, Magnin M, Garcia-Larrea L. 2016; Pain networks from the inside: spatiotemporal analysis of brain responses leading from nociception to conscious perception. Hum Brain Mapp. 37:4301–15. DOI: 10.1002/hbm.23310. PMID: 27391083. PMCID: PMC6867521. PMID: https://www.scopus.com/inward/record.uri?partnerID=HzOxMe3b&scp=84978194822&origin=inward.


26. Xu N, Tang XH, Pan W, Xie ZM, Zhang GF, Ji MH, et al. 2017; Spared nerve injury increases the expression of microglia M1 markers in the prefrontal cortex of rats and provokes depression-like behaviors. Front Neurosci. 11:209. DOI: 10.3389/fnins.2017.00209. PMID: 28458629. PMCID: PMC5394168. PMID: https://www.scopus.com/inward/record.uri?partnerID=HzOxMe3b&scp=85019059572&origin=inward.


27. Parvardeh S, Sabetkasaei M, Moghimi M, Masoudi A, Ghafghazi S, Mahboobifard F. 2018; Role of L-arginine/NO/cGMP/KATP channel signaling pathway in the central and peripheral antinociceptive effect of thymoquinone in rats. Iran J Basic Med Sci. 21:625–33. DOI: 10.22038/IJBMS.2018.26255.6438. PMID: 29942454. PMCID: PMC6015243. PMID: https://www.scopus.com/inward/record.uri?partnerID=HzOxMe3b&scp=85046283684&origin=inward.
28. Fan W, Huang F, Wu Z, Zhu X, Li D, He H. 2012; The role of nitric oxide in orofacial pain. Nitric Oxide. 26:32–7. DOI: 10.1016/j.niox.2011.11.003. PMID: 22138296. PMID: https://www.scopus.com/inward/record.uri?partnerID=HzOxMe3b&scp=83655172305&origin=inward.


29. Miranda HF, Noriega V, Sierralta F, Sotomayor-Zárate R, Aguilera V, Prieto JC. 2020; Nitric oxide mediated dexketoprofen antinociception. J Exp Res. 8:16–22.
30. Fakhri S, Ahmadpour Y, Rezaei H, Kooshki L, Moradi SZ, Iranpanah A, et al. 2020; The antinociceptive mechanisms of melatonin: role of L-arginine/nitric oxide/cyclic GMP/KATP channel signaling pathway. Behav Pharmacol. 31:728–37. DOI: 10.1097/FBP.0000000000000579. PMID: 32925224. PMID: https://www.scopus.com/inward/record.uri?partnerID=HzOxMe3b&scp=85095861401&origin=inward.


31. Mohammadi S, Fakhri S, Mohammadi-Farani A, Farzaei MH, Abbaszadeh F. 2021; Astaxanthin engages the l-arginine/NO/cGMP/KATP channel signaling pathway toward antinociceptive effects. Behav Pharmacol. 32:607–14. DOI: 10.1097/FBP.0000000000000655. PMID: 34561366. PMID: https://www.scopus.com/inward/record.uri?partnerID=HzOxMe3b&scp=85121948700&origin=inward.


32. Zhuo M, Meller ST, Gebhart GF. 1993; Endogenous nitric oxide is required for tonic cholinergic inhibition of spinal mechanical transmission. Pain. 54:71–8. DOI: 10.1016/0304-3959(93)90101-T. PMID: 8397374. PMID: https://www.scopus.com/inward/record.uri?partnerID=HzOxMe3b&scp=0027253732&origin=inward.


33. Iwamoto ET, Marion L. 1994; Pharmacological evidence that nitric oxide mediates the antinociception produced by muscarinic agonists in the rostral ventral medulla of rats. J Pharmacol Exp Ther. 269:699–708. PMID: 7514222. PMID: https://www.scopus.com/inward/record.uri?partnerID=HzOxMe3b&scp=0028245415&origin=inward.
34. Pan HL, Chen SR, Eisenach JC. 1998; Role of spinal NO in antiallodynic effect of intrathecal clonidine in neuropathic rats. Anesthesiology. 89:1518–23. DOI: 10.1097/00000542-199812000-00031. PMID: 9856728. PMID: https://www.scopus.com/inward/record.uri?partnerID=HzOxMe3b&scp=0032422242&origin=inward.


35. Kawabata A, Fukuzumi Y, Fukushima Y, Takagi H. 1992; Antinociceptive effect of L-arginine on the carrageenin-induced hyperalgesia of the rat: possible involvement of central opioidergic systems. Eur J Pharmacol. 218:153–8. DOI: 10.1016/0014-2999(92)90159-2. PMID: 1327824. PMID: https://www.scopus.com/inward/record.uri?partnerID=HzOxMe3b&scp=0026741334&origin=inward.


36. Roche AK, Cook M, Wilcox GL, Kajander KC. 1996; A nitric oxide synthesis inhibitor (L-NAME) reduces licking behavior and Fos-labeling in the spinal cord of rats during formalin-induced inflammation. Pain. 66:331–41. DOI: 10.1016/0304-3959(96)03025-4. PMID: 8880857. PMID: https://www.scopus.com/inward/record.uri?partnerID=HzOxMe3b&scp=0030220595&origin=inward.


37. Oh M, Lee W, Go Y. 2006; The antinociceptive effect of intraperitoneally administered nonselective nitric oxide synthase inhibitor on the rat formalin test. Korean J Pain. 19:142–5. DOI: 10.3344/kjp.2006.19.2.142.


38. Kampa M, Hatzoglou A, Notas G, Niniraki M, Kouroumalis E, Castanas E. 2001; Opioids are non-competitive inhibitors of nitric oxide synthase in T47D human breast cancer cells. Cell Death Differ. 8:943–52. DOI: 10.1038/sj.cdd.4400893. PMID: 11526449. PMID: https://www.scopus.com/inward/record.uri?partnerID=HzOxMe3b&scp=0034874425&origin=inward.


39. Dietis N, Rowbotham DJ, Lambert DG. 2011; Opioid receptor subtypes: fact or artifact? Br J Anaesth. 107:8–18. DOI: 10.1093/bja/aer115. PMID: 21613279. PMID: https://www.scopus.com/inward/record.uri?partnerID=HzOxMe3b&scp=79959423686&origin=inward.


40. Chung E, Burke B, Bieber AJ, Doss JC, Ohgami Y, Quock RM. 2006; Dynorphin-mediated antinociceptive effects of L-arginine and SIN-1 (an NO donor) in mice. Brain Res Bull. 70:245–50. DOI: 10.1016/j.brainresbull.2006.05.008. PMID: 16861110. PMID: https://www.scopus.com/inward/record.uri?partnerID=HzOxMe3b&scp=33745970186&origin=inward.


41. Javanmardi K, Parviz M, Sadr SS, Keshavarz M, Minaii B, Dehpour AR. 2005; Involvement of N-methyl-D-aspartate receptors and nitric oxide in the rostral ventromedial medulla in modulating morphine pain-inhibitory signals from the periaqueductal grey matter in rats. Clin Exp Pharmacol Physiol. 32:585–9. DOI: 10.1111/j.1440-1681.2005.04234.x. PMID: 16026519. PMID: https://www.scopus.com/inward/record.uri?partnerID=HzOxMe3b&scp=22544467758&origin=inward.


42. Hafeshjani ZK, Karami M, Biglarnia M. 2012; Nitric oxide in the hippocampal cortical area interacts with naloxone in inducing pain. Indian J Pharmacol. 44:443–7. DOI: 10.4103/0253-7613.99299. PMID: 23087502. PMCID: PMC3469944. PMID: https://www.scopus.com/inward/record.uri?partnerID=HzOxMe3b&scp=84865773679&origin=inward.


43. Pol O. 2007; The involvement of the nitric oxide in the effects and expression of opioid receptors during peripheral inflammation. Curr Med Chem. 14:1945–55. DOI: 10.2174/092986707781368469. PMID: 17691937. PMID: https://www.scopus.com/inward/record.uri?partnerID=HzOxMe3b&scp=34848885252&origin=inward.


44. Hervera A, Negrete R, Leánez S, Martín-Campos JM, Pol O. 2011; Peripheral effects of morphine and expression of μ-opioid receptors in the dorsal root ganglia during neuropathic pain: nitric oxide signaling. Mol Pain. 7:25. DOI: 10.1186/1744-8069-7-25. PMID: 21486477. PMCID: PMC3094254. PMID: https://www.scopus.com/inward/record.uri?partnerID=HzOxMe3b&scp=79953889325&origin=inward.


45. Cadet P, Mantione K, Bilfinger TV, Stefano GB. 2001; Real-time RT-PCR measurement of the modulation of Mu opiate receptor expression by nitric oxide in human mononuclear cells. Med Sci Monit. 7:1123–8. PMID: 11687719. PMID: https://www.scopus.com/inward/record.uri?partnerID=HzOxMe3b&scp=0035215298&origin=inward.
46. Babey AM, Kolesnikov Y, Cheng J, Inturrisi CE, Trifilletti RR, Pasternak GW. 1994; Nitric oxide and opioid tolerance. Neuropharmacology. 33:1463–70. DOI: 10.1016/0028-3908(94)90050-7. PMID: 7532830. PMID: https://www.scopus.com/inward/record.uri?partnerID=HzOxMe3b&scp=0028051645&origin=inward.


47. Heinzen EL, Booth RG, Pollack GM. 2005; Neuronal nitric oxide modulates morphine antinociceptive tolerance by enhancing constitutive activity of the mu-opioid receptor. Biochem Pharmacol. 69:679–88. DOI: 10.1016/j.bcp.2004.11.004. PMID: 15670586. PMID: https://www.scopus.com/inward/record.uri?partnerID=HzOxMe3b&scp=12744277419&origin=inward.
48. Huang L, Wyse BD, Williams CM, Smith MT. 2019; Nitric oxide modulates μ-opioid receptor function in vitro. Clin Exp Pharmacol Physiol. 46:676–85. DOI: 10.1111/1440-1681.13091. PMID: 30933370. PMID: https://www.scopus.com/inward/record.uri?partnerID=HzOxMe3b&scp=85064493111&origin=inward.


49. Cheriyan J, Sheets PL. 2018; Altered excitability and local connectivity of mPFC-PAG neurons in a mouse model of neuropathic pain. J Neurosci. 38:4829–39. DOI: 10.1523/JNEUROSCI.2731-17.2018. PMID: 29695413. PMCID: PMC6596022. PMID: https://www.scopus.com/inward/record.uri?partnerID=HzOxMe3b&scp=85049306424&origin=inward.


Fig. 1
The microinjection schedule and study timeline used in this study. On day 1, rats underwent spared nerve injury (SNI) surgery. On day 7, medial prefrontal cortex (mPFC) on both sides of the brain was cannulated. Paw withdrawal threshold fifty percentage and locomotor behavior were recorded on the 14th and 20th day, respectively. Intra-mPFC microinjections of L-arginine and antagonists were performed at 6 and 3 minutes before allodynia recording, respectively. Fifteen minutes before, and on 5, 25, 45 and 65 minutes after infusion of drugs mechanical allodynia was evaluated. On day 20, the brains were separated for tip of cannulas verification.
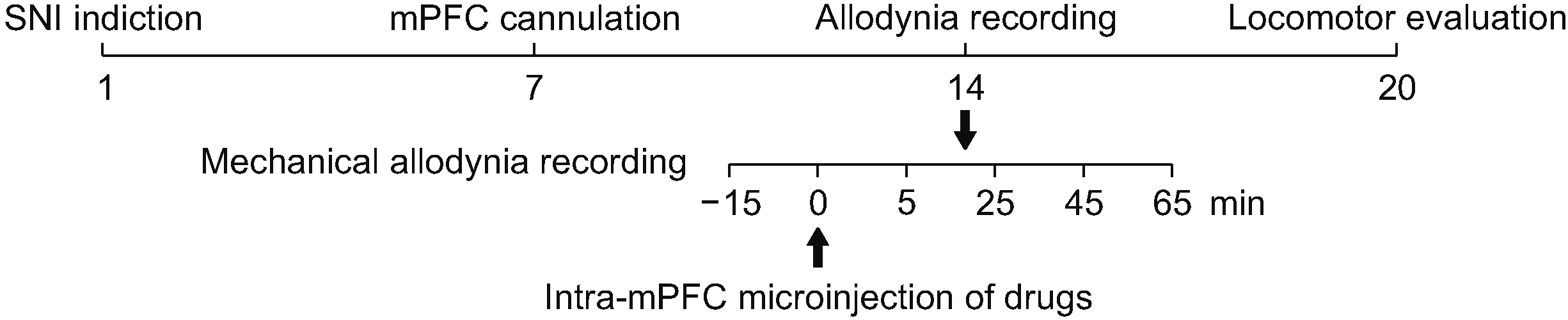
Fig. 2
Entry point of microinjection cannulas on the brain surface (white arrows, A) and the cannulas tip (white arrows, B, left) in the medial prefrontal cortex (mPFC). (B, right) shows the rat mPFC. Main sub-regions of the mPFC, the infralimbic (IL) and prelimbic (PrL) cortices have also been shown. Revised from Paxinos and Watson (The rat brain in stereotaxic coordinates; 2007) [20] with original copyright holder’s permission.
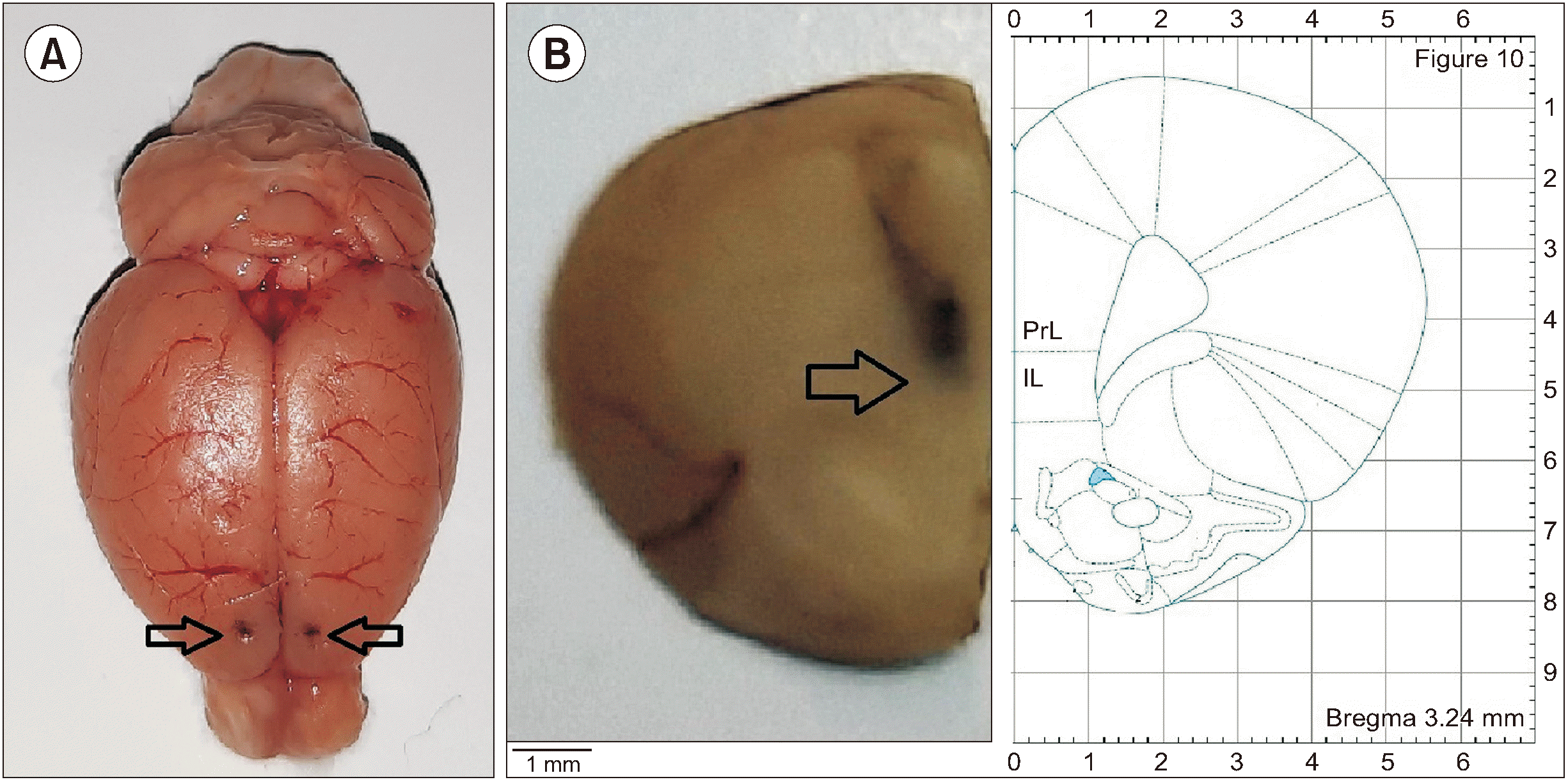
Fig. 3
The effects of normal saline (NS) and L-arginine (L-Arg) infusion into medial prefrontal cortex (mPFC) on spared nerve injury (SNI) induced mechanical allodynia. Values represent the mean ± SEM (n = 6 animals per group). A three-minute time interval before recordings of mechanical allodynia was used to microinject saline and L-Arg. (A) The paw withdrawal threshold fifty percent (50% PWT) change before and after drugs infusion. Different letters represent significant differences (P < 0.001, two way ANOVA and Bonferroni post hoc tests) for all time-points after microinjections of L-Arg at doses of 2.87, 11.5, and 45.92 nmol compared with NS group in SNI rats. (B) Related area under the curve (AUC) of mechanical allodynia after microinjection of NS and L-Arg. Different letters represent significant differences (P < 0.001) using one way ANOVA and Tukey’s post hoc tests. (C) Dose-response curve of L-Arg on SNI-induced allodynia. LA: L-Arg, SEM: standard error of the mean, ANOVA: analysis of variance.
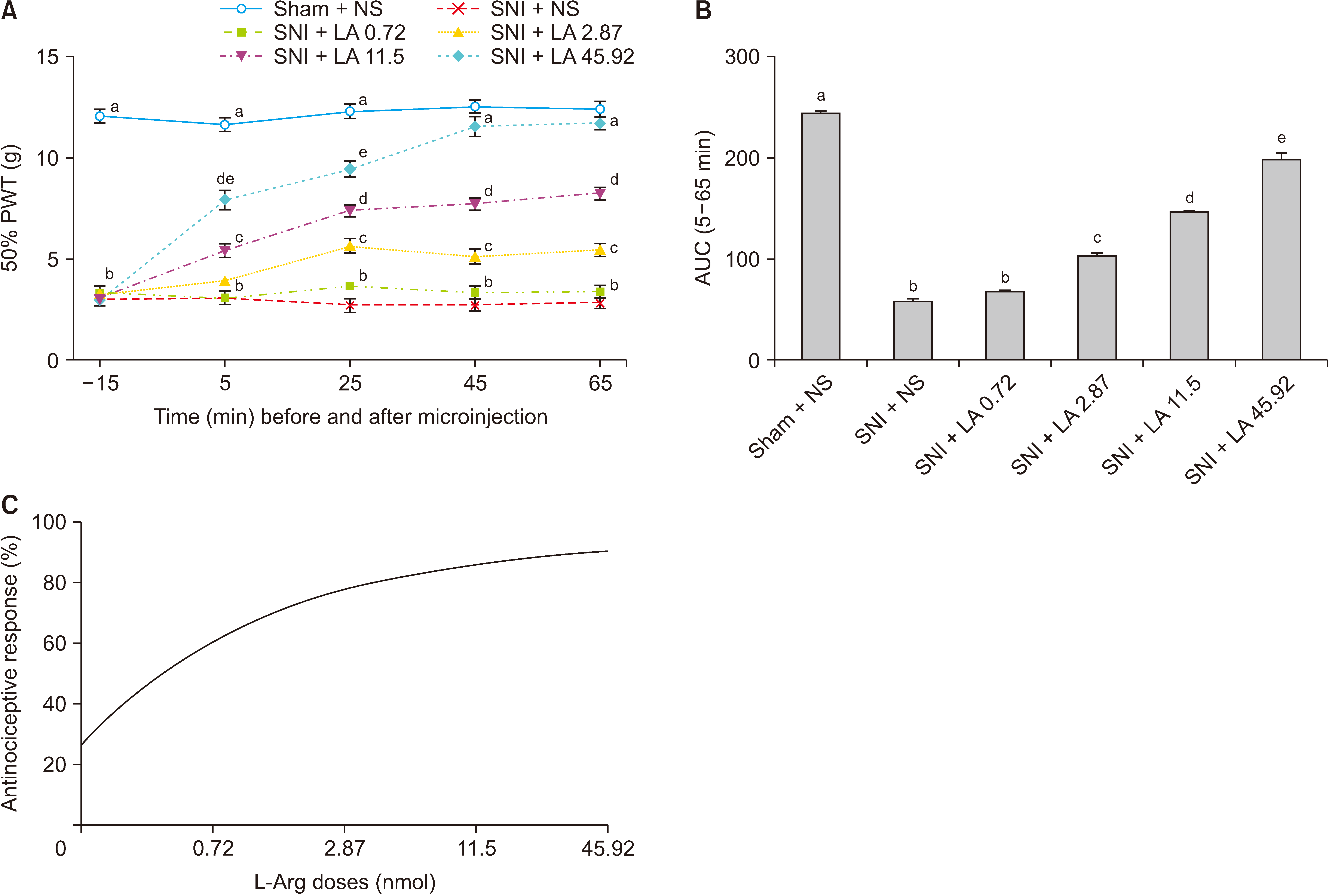
Fig. 4
The effects of N(gamma)-nitro-L-arginine methyl ester (L-NAME) alone, and prior to L-arginine (L-Arg, 45.92 nmol) microinjections into medial prefrontal cortex (mPFC) on spared nerve injury (SNI) induced mechanical allodynia. Values represent the mean ± SEM (n = 6 animals per group). Microinjection of L-Arg and L-NAME was performed 3 and 6 minutes before mechanical allodynia recordings, sequentially. (A) The paw withdrawal threshold fifty percent (50% PWT) change before and after drugs infusion. Different letters represent significant differences (P < 0.001, two way ANOVA and Bonferroni post hoc tests) for all time-points after microinjections of L-NAME (17.15 nmol) prior to L-Arg (45.92 nmol) compared with only L-Arg (45.92 nmol) treated group in SNI rats. (B) Related area under the curve (AUC) of mechanical allodynia after microinjection of normal saline (NS) and drugs. Different letters represent significant differences (P < 0.001) using one way ANOVA and Tukey’s post hoc tests. LA: L-Arg, SEM: standard error of the mean, ANOVA: analysis of variance.
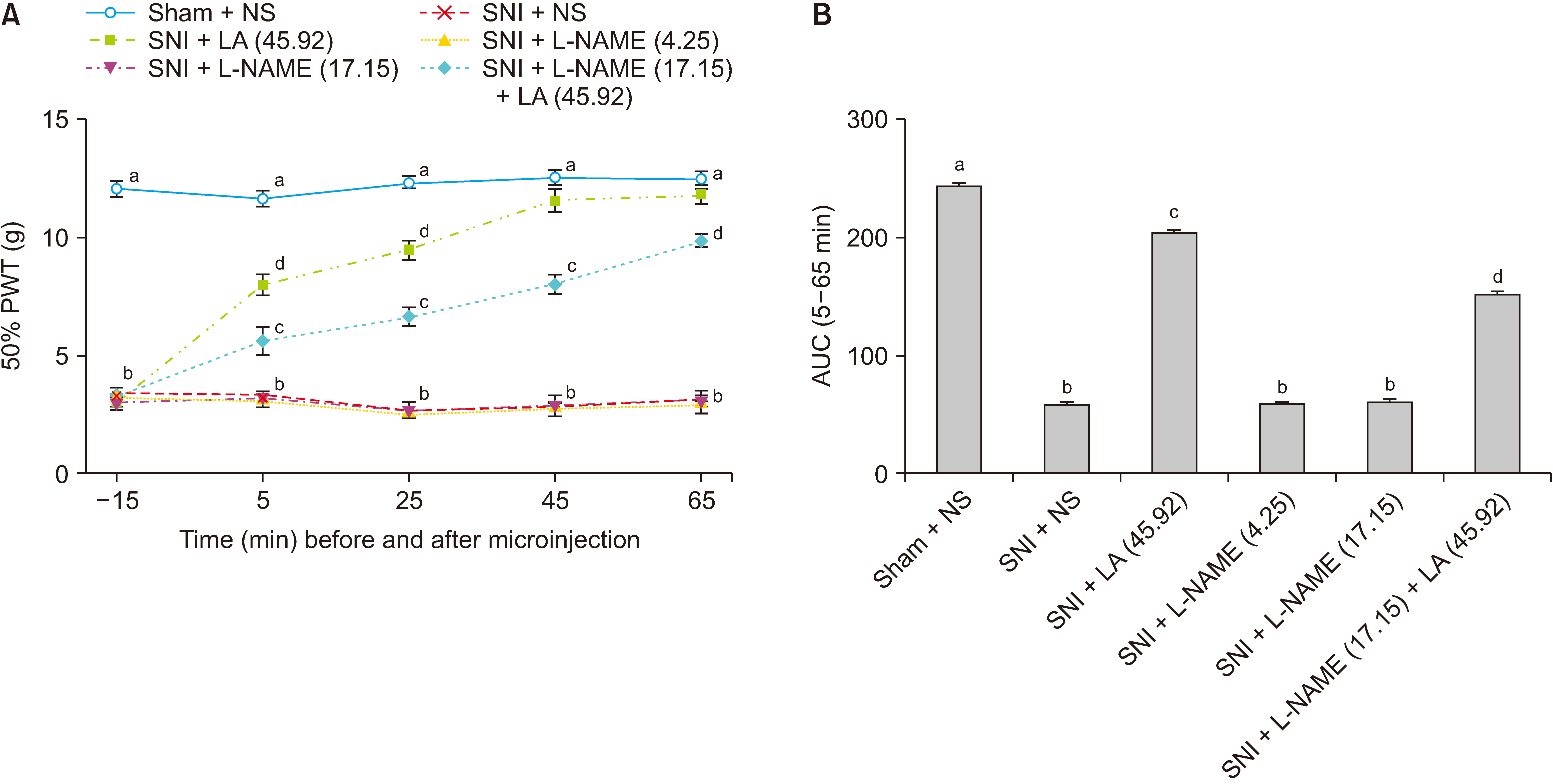
Fig. 5
The effects of naloxonazine (Nlz), naltrindole (Ntd), and nor-binaltorphimine (Nbt) microinjections alone, and prior to L-arginine (L-Arg, 45.92 nmol) into medial prefrontal cortex (mPFC) on spared nerve injury (SNI) induced mechanical allodynia. Values represent the mean with their mean ± SEM (n = 6 animals per group). Opioid antagonists and L-Arg were microinjected 6 and 3 minutes before mechanical allodynia recordings, respectively. (A–C) The paw withdrawal threshold fifty percent (50% PWT) change before and after drugs infusion. Different letters represent significant differences (P < 0.001, two way ANOVA and Bonferroni post hoc tests) for all time-points after microinjections of Nlz (1.54 nmol) prior to L-Arg (45.92 nmol) compared with only L-Arg (45.92 nmol) treated group in SNI rats. (D) Related area under the curve (AUC) of mechanical allodynia after microinjection of normal saline (NS) and drugs. Different letters represent significant differences (P < 0.001) using one way ANOVA and Tukey’s post hoc tests. LA: L-Arg, SEM: standard error of the mean, ANOVA: analysis of variance.
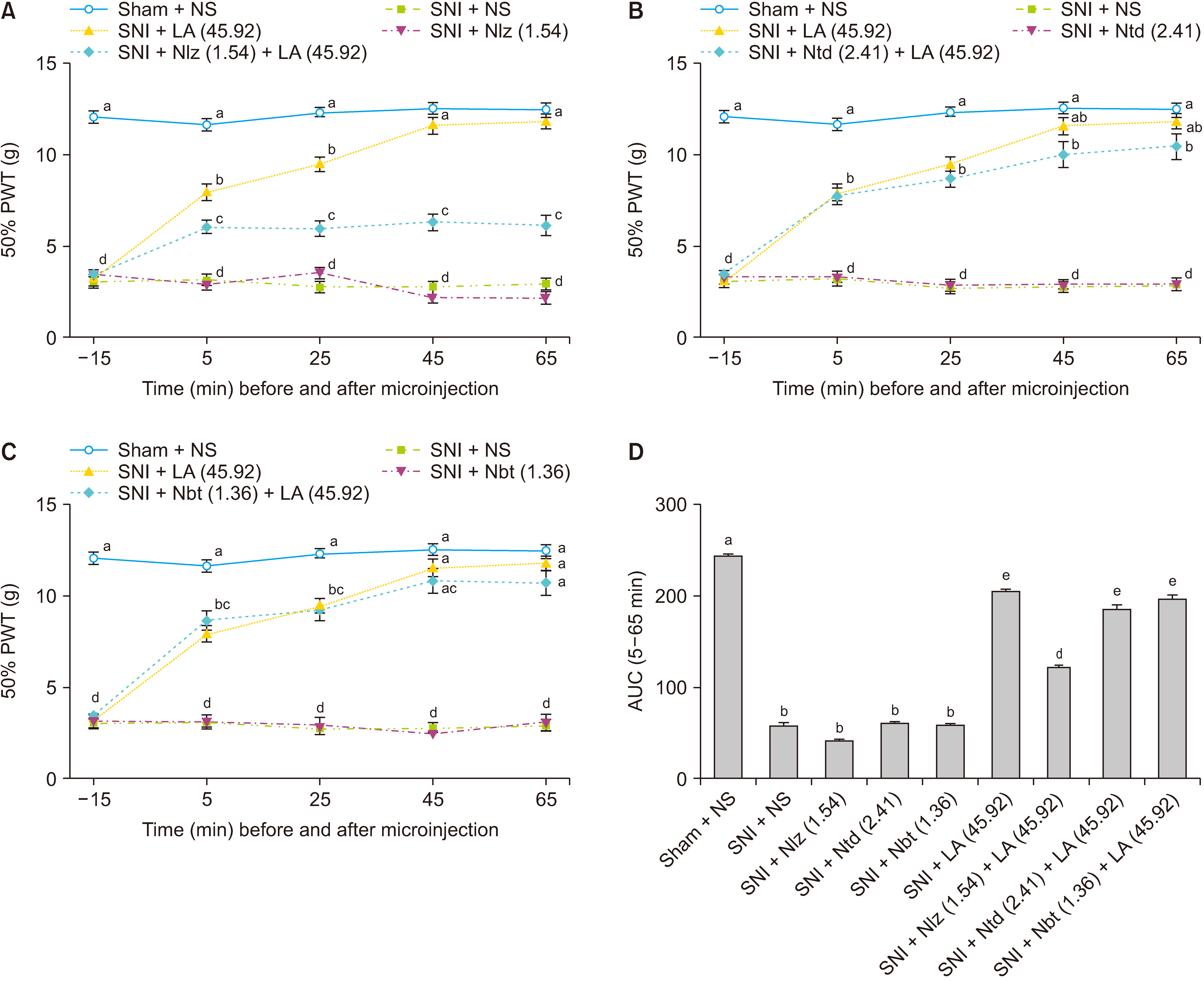
Fig. 6
Effects of intra-medial prefrontal cortex microinjection of normal saline (NS), L-arginine (L-Arg), N(gamma)-nitro-L-arginine methyl ester (L-NAME) and opioid antagonists on photo beam breaks number in spared nerve injury (SNI) rats. Values represent the mean ± SEM (n = 6 animals per group). Similar letters indicate no significant differences among groups (one way ANOVA and Tukey’s post hoc tests). LA: L-Arg, Nlz: naloxonazine, Ntd: naltrindole, Nbt: nor-binaltorphimine, SEM: standard error of the mean, ANOVA: analysis of variance.
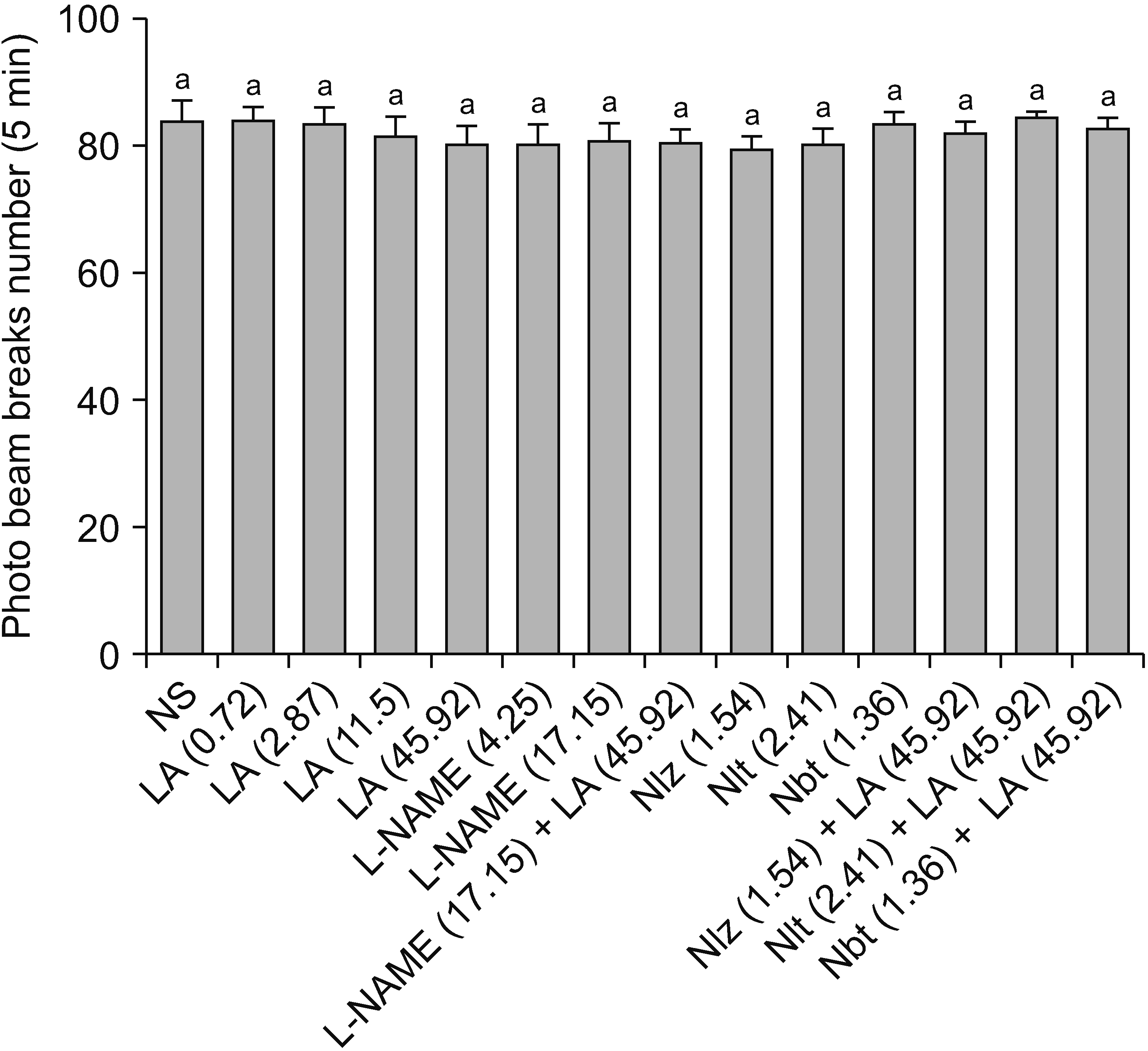