Abstract
A complex network of biochemical pathways carries out the process of muscle regeneration/growth following resistance exercise. The initial inflammatory response following muscle damage is primarily mediated by the nuclear factor κ-light-chain-enhancer of activated B cells (NF-κB), cyclooxygenase enzymes, and prostaglandins. Muscle damage also stimulates the activation, proliferation, differentiation, migration, and fusion of satellite cells onto damaged myofibers, resulting in myofibrillar hypertrophy. The progression of the myogenic lineage is predominantly coordinated by the wingless/integrated family of glycoproteins which engages in crosstalk with NF-κB and the mitogen-activated protein kinase (MAPK)/extracellular signaling-regulated kinase network. The MAPK cascade is essential for mechanotransduction, the process of converting mechanical stimuli into biochemical responses such as accelerated protein synthesis and satellite cell activation. Muscle protein synthesis is primarily governed by the insulin-like growth factor 1/phosphoinositide 3-kinase/protein kinase B/mammalian target of rapamycin pathway. Several calcium-dependent pathways are also integrated into the process of myogenesis and influence skeletal muscle plasticity. These dynamic interactions are part of the anabolic priming by resistance exercise effect, which defines resistance exercise as an acute catabolic event that potentiates multiple downstream anabolic pathways. Plateaus in muscle growth are attributed to deteriorating inflammatory signaling with repeated bouts of muscle damage as well as increasing thresholds for continuous adaptations, which ultimately become unreachable beyond a certain point. The physiological ceiling of skeletal muscle mass is also credited to myostatin. However, recent discoveries suggest the role of myostatin is not limited to preventing excessive skeletal muscle hypertrophy.
The benefits of resistance exercise such as enhanced insulin sensitivity, reduced morbidity, and healthy aging are well-established in literature1,2. Numerous hormones and biochemical pathways coordinate the adaptive myotropic response following resistance exercise3,4. These mechanisms are complex and compose both independent and synergistic vectors leading to skeletal muscle growth. This growth is primarily achieved via hypertrophy, the thickening of muscle fibers. It is proposed that such hypertrophic adaptations occur in response to mechanical tension, metabolic stress, and/or muscle damage5. Provided an adequate stimulus, skeletal muscle regeneration composes three phases: (1) an initial inflammatory phase, followed by (2) satellite cell activation and differentiation, and finally (3) a maturation phase where newly formed myofibers are remodeled6. Various attempts have been made to collaborate the numerous components of postnatal skeletal muscle development into a single comprehensive manuscript2,4,7-9. Although our previous reviews have described the mechanisms of muscle hypertrophy10, we believe there has yet to be a review outlining this process in proper sequence with respect to the dynamic interactions between endogenous metabolic routes and homeostasis. Further, there is significant overlap of molecular targets (i.e., mammalian target of rapamycin [mTOR]) and hormones (i.e., insulin-like growth factor 1 [IGF-1]) between skeletal muscle growth mechanisms and pathological growth states like cancer11,12. A well-rounded comprehension of such topics would prove useful in designing nutrition/training regimens for elite athletes, curing myopathies and disrupted growth diseases, and expanding the scope of knowledge regarding the human body as a whole. Hence, the purpose of our present review is to clearly illustrate the step-by-step process in which mechanical and biochemical stimuli from resistance exercise leads to contractile protein accretion and other adaptations (Fig. 1). Moreover, this review aims to elucidate tips for practical application based on the science of muscle growth, explain the underlying factors that limit excessive muscularity, and illuminate biological paradigms and topics for future research and development. To begin, we first establish the origin of new myonuclei, satellite cells.
Stem cells are undifferentiated progenitor cells capable of developing into one or multiple types of tissue-specific cells. Muscle-derived stem cells are multipotent and are thought to give rise to the immediate precursors to myoblasts, satellite cells13. Satellite cells are unipotent, meaning they can only differentiate into one type of cell, a myoblast, and are found between the basal lamina and sarcolemma of muscle fibers. In undamaged muscle tissue of adults, satellite cells are mostly quiescent14. Following muscle damage, the regeneration of muscle tissue can be attributed to the recruitment and myogenic commitment of satellite cells in concordance with a positive net protein balance13. This regeneration process is slightly overcompensated, accumulating to tangible muscle growth with consistent repeated bouts. Thus, the proper progression of the myogenic linage, which constitutes the activation, proliferation, differentiation, migration, and fusion of satellite cells, largely determines the rate and capacity of muscle growth. Satellite cells have the potential to either self-renew and replenish the satellite cell reserve pool or differentiate into myoblasts9,13,14. In the latter case, activated satellite cells undergo myogenic commitment and proliferation. After the proliferation phase, myoblasts develop into myocytes and can fuse together to generate myotubes8. Finally, the fusion of these newly formed myotubes to damaged myofibers, or the direct fusion of myoblasts to damaged myofibers, results in myofibrillar regeneration/hypertrophy (Section: Myofibrillar and Sarcoplasmic Hypertrophy)9. Therefore, it is widely accepted that the primary source of acquired myonuclei during muscle regeneration/growth is through the fusion of differentiated muscle stem cells to damaged muscle fibers either directly or as newly formed myotubes9. The myonuclear domain hypothesis suggests that there is a fixed ratio between the sarcoplasmic volume and the number of myonuclei4. Indeed, sufficient myonuclear density facilitates messenger RNA (mRNA) transcription and ribosome-mediated protein translation15. However, type II muscle fibers have demonstrated the ability to hypertrophy in the absence of myonuclear accretion16. Hence, the concept of a fixed myonuclear domain remains confounded. The phenomenon known as muscle memory describes how previously hypertrophied muscles that have atrophied from detraining regrow to their hypertrophied state considerably faster on subsequent bouts of training compared to initial bouts of training. It is suggested that this is due to the preservation of acquired myonuclei in inactive muscle tissue17. As such, the retention of myonuclei is a long-term adaptation that enhances prospective short-term growth. Additional sustained exercise-induced modifications, such as changes to the epigenome, may also be implicated in this phenomenon. Nonetheless, satellite cell recruitment appears to be crucial for both ongoing and upcoming muscle growth.
Satellite cells are a functionally heterogenous population comprising some designated to expanding/replenishing the satellite cell reservoir as well as those that are myogenically committed13. Paired box 7 (PAX7) is the myogenic identity expressed by all satellite cells and is needed to direct pluripotent stem cells toward the myogenic lineage in adults13. The progression of the myogenic lineage is orchestrated by myogenic regulatory factors (MRFs) which include myogenic factor 5 (Myf5), myoblast determination protein (MyoD), MRF4, and myogenin (MyoG)18. As transcription factors, MRFs bind to sequence-specific DNA elements in the promoter region of muscle genes during hypertrophy4. MyoD and Myf5 appear to play important roles in initiating differentiation13. Approximately 10% of satellite cells never express Myf5 during development and this subset of the population is thought to exist primarily to replenish the satellite cell reservoir18. Likewise, Myf5+ satellite cells that do not upregulate MyoD may exist transiently for the same purpose13. PAX7+/Myf5– cells are non-committed satellite stem cells while PAX7+/Myf5+ cells are committed satellite progenitor cells6. Following MyoD upregulation, MyoG aids in fulfilling the differentiation of myogenic progenitors8. The co-expression of MyoD and MyoG leads to the upregulation of MRF4 during the terminal stages of differentiation, which emerges as the predominant MRF in fully differentiated muscle18. Newly formed myofibers are marked by a central nuclei and the expression of developmental myosin heavy chains8. Once activated, satellite cells can undergo either symmetric or asymmetric cell division. Symmetric cell division of stem cells generates two identical daughter cells is a crucial process to expand/preserve the stem cell population. On the other hand, asymmetric cell division generates one myogenically-committed apical daughter cell and one basal daughter cell pledged to self-renewel19. Myogenically-committed daughter cells subsequently undergo bouts of symmetric cell division as part of proliferation preceding differentiation19. The rates of symmetric and asymmetric satellite cell division, as well as coordination of proliferation and differentiation, must be precisely controlled, as imbalances may lead to depletion of the satellite cell reservoir and/or diminished muscle regeneration capacity. Signaling molecules that promote differentiation often inhibit proliferation, and vice versa. For example, quiescent/proliferating satellite cells express high levels of Notch, which prevents precocious differentiation and excessive depletion of the stem cell pool8,14. For differentiation to occur, canonical Wnt signaling antagonizes Notch, and this can only occur after adequate proliferation has taken place (Section: The Wnt Signaling Pathways)8. Evidently, not only the progression but also the proper sequence of the myogenic lineage is necessary for adequate muscle regeneration.
In adults, the significant growth of skeletal muscle in response to mechanical overload is primarily attributed to muscle fiber hypertrophy20. The gain in the number of muscle cells is termed hyperplasia. Evidence for the occurrence of hyperplasia in adults is limited, although it is speculated that new muscle fibers can be acquired via satellite cell proliferation and longitudinal fiber splitting21. On the contrary, skeletal muscle hypertrophy has been well documented in literature and can be further classified as either myofibrillar or sarcoplasmic hypertrophy. In myofibrillar hypertrophy, as previously discussed, satellite cells fuse to damaged myofibrils, donating their intracellular proteins and nuclei to generate new contractile elements, termed sarcomeres, to regenerating muscle tissue4. The parallel addition of sarcomeres during myofibrillar hypertrophy results in an increased cross-sectional area of a muscle and a correspondingly greater contractile strength4. Conversely, sarcoplasmic hypertrophy refers to adaptations enhancing the volume of the sarcolemma and/or sarcoplasm accompanied by an increase in mitochondrial volume, sarcoplasmic reticulum, t-tubules, enzymes, and/or substrate (i.e., glycogen) content22. Notably, this type of hypertrophy appears to play a significant role in type I muscle fibers as oxidative muscle fibers maintain higher resting intracellular Ca2+ levels and mitochondrial content than their glycolytic counterparts (Section: Calcium as a Regulator of Muscle Size and Plasticity)23. Along the spectrum of muscle fiber types, glycolytic fibers generally maintain a higher propensity for hypertrophy overall as there is an inverse relationship between striated muscle fiber size and its oxidative capacity24. During exercise, particularly high-volume/short-rest exercise, metabolites (i.e., lactate and hydrogen ions) accumulate inside of the working muscle, generating an influx gradient of extracellular fluid into the sarcoplasm25. These metabolites also cause vasodilation and capillary recruitment, resulting in a transiently thicker muscle that can best be described as a swelling of the muscle and this phenomenon is often referred to as the muscle pump26. Therefore, the primary influential factor to skeletal muscle perfusion is its metabolic rate26. Training-induced edema, active hyperemia, and skeletal muscle perfusion are all phrases synonymous with the muscle pump5,27. High-volume/hyperemia-focused blood flow restriction training has shown to induce sarcoplasmic hypertrophy25. Similar training styles popular among bodybuilders induces capillary neoformation (angiogenesis) which likely facilitates prospective bouts of skeletal muscle perfusion7,27. In essence, sarcoplasmic hypertrophy results in a larger muscle cross-sectional area, but not necessarily a concomitant increase in muscle strength because it does not comprise the addition of contractile elements22. Resistance exercise rapidly stimulates a sustained elevation in muscle protein synthesis (MPS) favoring myofibrillar hypertrophy28. However, sarcoplasmic hypertrophy concomitant with resistance exercise appears to complement the acquisition of myofibrillar hypertrophy7.
In the sarcoplasm expansion hypothesis of hypertrophy proposed by Roberts et al.27, sarcoplasmic hypertrophy induces spatial priming of muscle fibers, an optimal preliminary step to myonuclear accretion and subsequent myofibrillar hypertrophy. Likewise, Vann et al.29, describe how an expansion of the sarcoplasm facilitates the accretion of new contractile proteins by assuring sufficient cellular space. Schoenfeld and Contreras5 speculate that cellular swelling is perceived as a threat to the cell membrane’s integrity and results in adaptive responses such as satellite cell recruitment. Further, an increased amount of intramyocellular fluid caused by skeletal muscle perfusion likely facilitates nutrient uptake in damaged myofibers due to an amplified influx gradient. These concepts fortify the ability of sarcoplasmic hypertrophy/acute bouts of active hyperemia to complement myofibrillar hypertrophy and is in line with the anabolic priming effect of resistance exercise on muscle tissue effectuated by multiple stress and damage-sensitive pathways that potentiate downstream anabolic targets of muscle regeneration/growth (Section: Anabolic Priming by Resistance Exercise). Beyond neural adaptations, given the objective to maximize myofibrillar hypertrophy and strength, it would be germane to incorporate eccentric resistance training since eccentric contractions are associated with significant myofibrillar damage30. Thus, numerous models of skeletal muscle regeneration are based off eccentric exercises30-32. On the other hand, those prioritizing metabolic adaptations would benefit from hyperemia-focused workouts with short-rest intervals that maximize sarcoplasmic hypertrophy7. For competitive body-builders, both myofibrillar and sarcoplasmic hypertrophy are recognized as the primary goal is to maximize muscle size/development overall. Thus, the training style of many successful bodybuilders incorporates proper volume, intensity, and rest with various types of contractions. Beyond sarcoplasmic and myofibrillar hypertrophy, a third type of hypertrophy is described in the connective tissue of muscle where the volume of extracellular matrix expands and is accompanied by increased mineral and protein content20. This adaptation may serve an integral role in maintaining tendon integrity to match the increasing peak tensile load that can be generated by growing contractile fibers. At any rate, long-term muscle growth is the product of many short-term adaptations and the rate and capacity of muscle growth due to resistance training is largely dependent on the ability of acute inflammatory responses to stimulate downstream anabolic pathways. The fundamental mechanisms of resistance exercise-induced inflammation are outlined below.
Inflammation is the general physiological reaction to any type of tissue damage. As such, the regenerative processes following a bout of resistance exercise involve an array of coordinated immune responses. In addition to their role in removing cell debris (i.e., damaged muscle proteins), leukocytes recruited to the site of tissue damage release proinflammatory cytokines. However, since prolonged inflammation impedes longer-acting downstream effectors of MPS such as mTOR (Section: The Mechanistic/Mammalian Target of Rapamycin), one should aim to maximize acute/local inflammation while minimizing chronic/systemic inflammation33. In this respect, proinflammatory monocytes recruited to the site of skeletal muscle injury transform shortly after into anti-inflammatory macrophages to support optimal myogenesis34. Further, these recruited macrophages release growth factors that stimulate myogenic precursor cells34. Disruption of the acute and localized inflammatory signal in response to muscle damage hinders muscle growth (Section: Depletion of Arachidonic Acid in Trained Athletes).
Among the various proinflammatory cytokines released, prostaglandins function as chief mediators of proper muscle regeneration35. Prostaglandins are lipids derived from ω-3 and ω-6 fatty acids and their synthesis begins with the cleavage of arachidonic acid (AA) from the phospholipid membrane by phospholipase A236. Prostaglandins cannot be stored inside cells and must be synthesized on-demand and therefore, the terminal prostaglandin product of this pathway is highly responsive to the environment. Appropriately, stimulators of AA cleavage and subsequent prostaglandin synthesis include mechanical stimulation, cellular damage, and/or stretch-stress37. Once liberated, AA is converted into prostaglandin H2 (PGH2), the precursor to all prostaglandins. PGH2 can be transformed into the vasoconstrictor and platelet aggregator, thromboxane A2, or one of four bioactive prostaglandins: prostaglandin E2 (PGE2), prostacyclin (PGI2), prostaglandin D2 (PGD2), and prostaglandin F2-α (PGF2-α)37. Each of these prostaglandins maintain unique and overlapping functions and are essential in the progression of the myogenic lineage. The biotransformation of AA into prostaglandins is mediated by cyclooxygenase (COX) enzymes which exist in two isoforms: COX-1 and COX-2. In the regulation of skeletal muscle turnover in relation to muscle injury, COX-2–derived prostaglandins emerge as crucial autocrine/paracrine factors35,38.
Early in the process of muscle regeneration, proliferating myoblasts and leukocytes generate large quantities of PGE2 and PGF2-α. These two prostaglandins have been proposed to influence nitrogen balance by increasing the rates of protein degradation and synthesis, respectively39. In this sense, PGE2 may seem detrimental to muscle growth; however, some proteolytic processes such as autophagy (the degradation and removal of unnecessary and/or damaged components) remain necessary to optimize long-term cellular functions40. Moreover, both PGF2-α and PGE2 are required for stretch-induced proliferation of myoblasts and PGE2 enhances myogenic differentiation supported by upregulated MyoD and MyoG expression41,42. Likewise, PGI2 promotes myotube formation and PGF2-α also assists in the engraftment of satellite cells onto damaged myofibers, interacts with the phosphoinositide 3-kinase (PI3K)/Akt/mTOR pathway to increase MPS (Section: The Phosphoinositide 3-Kinase/Akt/Mammalian Target of Rapamycin Pathway), and increases intracellular levels of calcium ions to activate nuclear factor of activated T cells (NFAT)37. The exact role of PGD2 in adult myogenesis has yet to be uncovered, though one of its metabolites is known to interact with nuclear factor κ-light-chain-enhancer of activated B cells (NF-κB) (Section: Nuclear Factor κ-Light-Chain-Enhancer of Activated B Cells) and peroxisome proliferator-activated receptor-γ36. Transient antimyogenic effects of PGD2 may serve as a deterrent for premature myogenesis during the early stages of inflammation and thus, PGD2 may serve an overall significant role in muscle growth37.
Nonsteroidal anti-inflammatory drugs (NSAIDs) inhibit COX enzymes and the production of downstream prostaglandins to elicit their anti-inflammatory effects. Several studies report that high doses and/or prolonged use of glucocorticoids and NSAIDs can interfere with resistance exercise-induced muscle regeneration/growth32,37. However, there is some discrepancy on the effects of NSAIDs on skeletal muscle adaptations to exercise, particularly regarding whether NSAIDs are overall beneficial or detrimental to long-term muscle growth. Beyond sample heterogeneity, potential differences in basal inflammatory states and treatment durations are proposed to account for these discrepancies38. Nonetheless, acute and localized inflammation appears to be necessary for maximizing resistance exercise adaptations. Inhibition of AA-derived inflammatory prostaglandin production via pharmacological blockade of COX enzymes has shown to completely obstruct MPS 24 hours following eccentric exercise31. On the other hand, many cachexic diseases stem from uncontrolled chronic/systemic inflammation while the supplementation of AA has shown to enhance skeletal muscle growth and aerobic capacity31,35,43. Although acute inflammation serves as an essential signal for repair, uncontrolled/prolonged inflammation will inevitably impede muscle regeneration/growth33. In trained athletes, the depletion of AA/prostaglandins appears to contribute to the stagnation of muscle growth as this results in diminishing acute inflammatory responses following muscle damage. Strength training is associated with a lower the phospholipid ω-6 (generally proinflammatory) to ω-3 (generally anti-inflammatory) ratio31. This matches the relationship between regular exercise and reduced resting systemic inflammation as well as the gradual amelioration of delayed-onset muscle soreness following repeated bouts of consistent exercise44,45. As the body becomes accustomed to exercise, the ability to generate a localized inflammatory response to repeated bouts of muscle damage deteriorates. Correspondingly, the threshold of anabolic stimulus (i.e., exercise volume and intensity) needed to cause further growth of muscle tissue increases, eventually beyond reach. Hence, the rate of new adaptations slows down and progress stagnates.
The NF-κB is a nuclear transcription factor that regulates over 150 genes associated with inflammation, redox status, and apoptosis2. NF-κB activation constitutes two classes: canonical and noncanonical. Canonical activation of the NF-κB pathway has shown to mediate the regeneration of skeletal muscle after injury by promoting the survival and proliferation of satellite cells and simultaneously preventing premature differentiation46. Upon differentiation, activation of the noncanonical NF-κB pathway enhances myoblast fusion46. NF-κB lies both upstream and downstream of key inflammatory mediators such as COX enzymes and prostaglandins, hence the early activation of exercise-induced inflammation is largely under the control of NF-κB47. Appropriately, metabolic stress and muscle damage during resistance exercise leads to the activation of NF-κB and like its physiological effect, inflammation, acute and local stimulation of NF-κB promotes muscle growth and regeneration by inducing proper progression of the myogenic lineage2,46. Conversely, chronic stimulation of this pathway often leads to muscle wasting and is involved in the cachexia of chronic inflammatory diseases44,48. Furthermore, constitutive activation of the canonical NF-κB pathway deters skeletal muscle regeneration46. This is likely because NF-κB also upregulates atrogenes (i.e., muscle RING-finger protein-1 [MuRF1]) associated with the ubiquitin-proteosome system48. To maximize muscle growth, precise activation of NF-κB is key.
Crosstalk between NF-κB and the Wnt pathways modifies satellite cell polarity, motility, and migration49,50. It is apparent that the balance between satellite cell proliferation and differentiation must be finely regulated, as an imbalance in the myogenic lineage can lead to discrepancies in muscle regeneration and/or depletion of the satellite stem cell pool. Appropriately, the homeostatic proportion of satellite cells remaining in the reserve pool versus those that are myogenically-committed is finely tuned by a highly conserved and efficient signaling cascade known as the Wnt signaling pathways51. Wnt members are a family of cysteine-rich glycoproteins that are involved in ensuring proper satellite cell proliferation and differentiation52. Two classes of Wnt signaling pathways have been described: the classical/ canonical and the noncanonical. The canonical pathway is also known as the Wnt/β-catenin pathway since it involves the β-catenin protein. On the other hand, the noncanonical Wnt pathways can be further classified into the Wnt/planar cell polarity (PCP) pathway and the Wnt/Ca2+ pathway50. The various Wnt pathways function synergistically to coordinate the progression of the myogenic lineage. Wnt/Fzd signaling pathways begin with the activation of the G protein-coupled receptor, frizzled (Fzd) by Wnt6,53. Downstream effectors of Wnt include the various calcium-dependent pathways; c-Jun N-terminal kinase (JNK), a constitute of the mitogen-activated protein kinase (MAPK)/extracellular signal-regulated kinase (ERK) cascade (Section: Mitogen-Activated Protein Kinase/Extracellular Signal-Regulated Kinase); and the PI3K/Akt/mTOR pathway51,54. Preceding differentiation and fusion, satellite cells first require adequate proliferative and migrational capacity which is mainly established by the noncanonical Wnt pathways54.
Cytoskeletal organization is a marker of cell polarity. In turn, cell polarity largely dictates its adhesive, migrational, and mitotic properties55. The Wnt/PCP pathway establishes polarized cellular structures and alters cytoskeletal organization, partially through its interaction with JNK6. As discussed before, both symmetrical and asymmetrical cell division are essential in generating sufficient myogenically-committed satellite cells. To regulate this balance, multiple Wnt subtypes maintain unique functions in myogenesis. Following muscle injury, Fzd7 and Wnt7a expression are upregulated in muscle tissue/satellite cells6. The activation of Fzd7 by Wnt7a preferentially drives symmetric cell division of satellite cells, a process carried out by the Wnt/PCP pathway56. Wnt7a also preferentially activates MyoD via the Wnt/Ca2+ pathway, whereas Wnt1 enhances Myf5 expression via the canonical Wnt pathway18. The Wnt/Ca2+ pathway also regulates cytoskeleton organization and cellular adhesion53. Wnt/Ca2+ signaling involves the activation of phospholipase C and Ca2+ influx, causing downstream stimulation of Ca2+-sensitive protein kinase C (PKC), calmodulin-dependent protein kinase (CaM-kinase II), and NFAT (Section: Calcium as a Regulator of Muscle Size and Plasticity)53. The Wnt signaling network is implicated in numerous crosstalk interactions, both within the various Wnt signaling pathways and beyond. For example, the activation of the Fzd by Wnt can lead to the activation of the Gsα subunit, which subsequently activates the PI3K/Akt/mTOR pathway54. Further, Wnt/Ca2+ activity can antagonize canonical Wnt signaling53. In myogenesis, these interactions preserve the proper orchestration of satellite cell activation.
Notch signaling prevents precocious differentiation by suppressing MyoD expression and retaining satellite cells in the quiescent state8. This effect primes the expression of target genes for subsequent activation19. A gradual switch from Notch to canonical Wnt signaling occurs throughout the activation of satellite cells following muscle damage to permit differentiation8,57. In the absence of Wnt, a complex formed by axin, adenomatous polyposis coli, and glycogen synthase kinase 3-β (GSK3-β) phosphorylates β-catenin, the marker of canonical Wnt/Fzd signaling, and inhibits its activity54. In the canonical Wnt/Fzd signaling pathway, the activation of Fzd by Wnt disassociates β-catenin from its inhibitory complex, which allows β-catenin to accumulate inside the cytoplasm52,53. Activated β-catenin then translocates into the nucleus where it guides the transcription of Wnt/β-catenin target genes. Notable Wnt/β-catenin targets include growth-control genes such as c-Myc and cyclin D1 that are key regulators of proliferation, thus β-catenin liberation supports noncanonical Wnt/Fzd pathways in the early steps of myogenic commitment49,58. Wnt/β-catenin signaling also promotes cell-to-cell adhesion, an imperative process for myotube formation/fusion, and stimulates transcriptional elongation, a component of MPS58. The liberation of β-catenin from its inhibitory complex also constitutes the inhibition of GSK3-β, which otherwise hinders mTOR-mediated MPS (Section: The Phosphoinositide 3-Kinase/Akt/Mammalian Target of Rapamycin Pathway)11,51. Therefore, the noncanonical and canonical arms of the Wnt/Fzd signaling network maintain overlapping functions; however, each pathway also exhibits unique fortes in progressing the myogenic lineage.
Both aerobic and resistance exercise result in acute increases of growth hormone (GH)3,25. Many of the metabolic effects of GH, especially those that are growth-promoting, are mediated through IGF-1, which is secreted by the liver in response to GH3. IGF-1 isoforms are also locally produced by extrahepatic tissues (i.e., myofibers) and recruited macrophages following resistance exercise3,59. The bioactivity of IGF-1 is regulated by IGF-binding proteins, which stabilize circulating IGF-1’s while also providing tissue-specific bioavailability60. IGF-1 receptors are present in activated satellite cells and adult myofibers4. Free IGF-1 triggers skeletal muscle hypertrophy by interacting with multiple signaling pathways, namely the PI3K/Akt/mTOR and MAPK/ERK pathways61-63. Initial translation of the IGF1 gene generates a pre-pro-peptide composed of an N-terminal signal peptide, mature IGF-1, and a C-terminal E-peptide extension63. Removal of the N-terminal signal peptide yields pro-IGF-1, which can then be further cleaved into mature IGF-1 and an E-peptide63. As such, alternative mRNA splicing of the IGF1 gene permits the formation of multiple pro-IGF-1 isoforms distinguished by their E-peptide extensions. Three pro-IGF-1 isoforms have been described in humans: IGF-1Ea, IGF-1Eb, and IGF-1Ec/mechano growth factor (MGF). The exact biological functions of these pro-IGF-1 isoforms have yet to be determined; however, it appears that the three pro-IGF-1 isoforms collaborate with one another as well as with mature IGF-1s to carry out the adaptive and regenerative response to resistance exercise64. Evidently, unique and overlapping functions across multiple subpathways of a major biochemical pathway is a recurring theme in resistance exercise physiology.
The correlation between the sequence of biological events following resistance exercise and the specific expression pattern of different pro-IGF-1 isoforms has led to the proposition of the MGF hypothesis. IGF-1Ea resides as the dominant pro-IGF-1 isoform and main precursor of mature IGF-1; however, resistance exercise results in the preferential upregulation of MGF transcription, and thus, MGF is the first pro-IGF-1 isoform to be upregulated in the process of myogenesis64,65. In healthy and physically active, but untrained men, MGF levels peaked first and demonstrated a transient increase 2 days following a maximal eccentric exercise protocol of knee extensor muscles. IGF-1Ea and IGF-1Eb exhibited similar expression profiles to one another and both were elevated beyond baseline at 5 days postexercise65. The Notch/Wnt antagonistic relationship was previously discussed, where only one process, proliferation or differentiation, is maximized at a given time8. This paradigm is demonstrated once again in the MGF hypothesis. According to the MGF hypothesis described by Matheny et al.66, the initial rise in MGF activates quiescent satellite cells and promotes proliferation of myogenic precursor cells but opposes differentiation. Following substantial proliferation, MGF levels decline and succeeding pro-IGF-1s commence differentiation66. Concurrently, mature IGF-1s generated from pro-IGF-1s potentiate several anabolic pathways (i.e., PI3K/Akt/mTOR) and promote early myogenic commitment of stem cells, while IGF-2 aids in the process of completing differentiation67. The MGF hypothesis is further supported by the findings of Ascenzi et al.64, where the proliferative effects of mouse IGF-1Eb (the rodent equivalent of human MGF) and the differentiative effects of IGF-1Ea in mice were demonstrated. It has been suggested that E-peptides, particularly the E-peptides of MGFs, act as mitogens to induce anabolic effects independently as well as complement the direct actions of pro-IGF-1s and mature IGF-1s65. Brisson and Barton63 reported the ability of E-peptides to increase phospho-ERK1/2, but not phospho-Akt in myoblasts, suggesting that E-peptides enhance the ability of IGF-1 to activate its receptor by enhancing the MAPK/ERK pathway but not the PI3K/Akt/mTOR pathway.
Two to three hours after a single bout of resistance exercise, a 40%–150% increase in MPS above baseline can be detected40,68. This elevation in MPS is sustained for up to 48 hours and is calibrated by multiple signaling pathways68. The NF-κB/COX/prostaglandin, Wnt/Fzd, and IGF-1 axes all intersect at the master nexus of anabolism: the mechanistic/mTOR which is a member of the PI3K-related kinases family12,49,69. As such, mTOR is regarded as one of the primary junctions between anabolic stimuli and their tangible effects on protein synthesis/degradation. Both mTORC1 and mTORC2, the two catalytic subunits of mTOR, have regulatory functions on cell proliferation and metabolism; however, mTORC1 appears to have a more direct influence on MPS while mTORC2 mainly influences cell survival/metabolism and cytoskeletal organization11. Crosstalk between mTORC1 and mTORC2 allows for an intricate feedback system involving multiple targets of the mTOR signaling network70. Activation of the mTOR/S6 kinase (S6K) pathway, which can be effected by insulin, hinders the ability of insulin to continuously activate through insulin receptor substrate protein-1 (IRS-1), resulting in insulin resistance and negative feedback of the insulin/IRS/mTOR axis11,71. Further, mTORC1 activates growth factor receptor bound protein 10, a negative regulator of the insulin/IGF-1 receptor, and S6K induces the degradation of IRS-112. This feedback system emerges as a cardinal factor to consider when determining optimal meal distribution patterns with respect to insulin sensitivity and maximizing MPS (Sections: Balancing Muscle Protein Synthesis and Autophagy and Optimal Meal Distribution to Maximize Muscle Protein Synthesis). An essential role of mTORC2 is stimulating mTORC1 through its activation of Akt12. In addition to mTORC2 and insulin, amino acids (mainly arginine and leucine), and several growth factors, including IGF-1, can activate mTORC1, while hypoxia, glucose deprivation, stress, and DNA damage inhibit mTORC112. Few studies show that resistance exercise-induced mTORC1 activation and the subsequent hypertrophy that occurs can be obtained without growth factor-mediated anabolic signaling62. This phenomenon likely stems from feedback-mediated compensations in mTOR signaling. However, as potent stimulators of mTOR, growth factors, particularly IGF-1, are likely needed to optimize all major growth pathways and elicit a maximal MPS response to resistance exercise. As an anabolic signaling pathway, mTOR requires energy to function. The energy status of cells is chiefly monitored by AMP-activated protein kinase (AMPK), which is activated by nutrient deprivation and some inflammatory cytokines (i.e., interleukin-6); thus, exercise and fasting activate AMPK68,72. Among the several AMPK isoforms, exercising skeletal muscle predominantly upregulates the AMPK α2β2γ3 type complex73. In general, AMPKs promote energy-conserving processes such as glucose uptake and mitochondrial biogenesis while suppressing energy-spending processes such as mTORC1-mediated MPS68. Upon exercise cessation and given an abundance of nutrients (i.e., from a post-workout meal), AMPK signaling declines and MPS is enabled via the Akt/mTOR pathway. This process is aided by the postexercise IGF-1 and GH surge3. Key myogenic genes involved in satellite cell proliferation and differentiation (i.e., PAX7 and MRFs) are also influenced by mTOR74.
The mTOR signaling network involves a complex series of activations, inhibitions, and disinhibitions. One route to mTORC1 activation starts with the stimulation of PI3K by growth factors. PI3K then activates Akt, also known as protein kinase B75. Subsequently, Akt inhibits negative regulators of mTORC1 such as proline-rich Akt substrate-40 and tuberous sclerosis complex (TSC), allowing mTORC1 to induce the anabolism of proteins, lipids, and nucleic acids through its downstream effectors72. p70 ribosomal protein S6K1 and eukaryotic translation initiation factor 4E (eIF4E)-binding protein (4E-BP1) are the two primary substrates of mTORC111,75. Phosphorylation of 4E-BP1 by mTORC1 inhibits the sequestration of translation initiation factor, eIF4E, promoting cap-dependent mRNA translation70. Phosphorylation of S6K1 by mTORC1 also promotes cap-dependent mRNA translation as well as mRNA biogenesis70. Beyond mTORC1 stimulation, Akt maintains additional functions in the process of myogenesis. Some of these functions include Akt’s ability to enhance cellular adenosine triphosphate content, mediate the positive effects of IGF-1 in skeletal muscle, and promote glycogen synthesis by inhibiting GSK3-β69. Akt also prevent muscle protein breakdown (MPB) by suppressing the expression of forkhead box O (FOXO) atrogenes such as MuRF1, atrogin1, and regulated in development and DNA damage responses 169,72. Furthermore, autophagy and apoptosis are inhibited by mTORC1 and Akt, respectively11,12. Autophagy and apoptosis are biological processes crucial to an organisms health and longevity. Thus, this raises the following question: How does one most effectively allocate periods of anabolism (i.e., MPS) and catabolism (i.e., autophagy)?
Acute suppression of catabolism in concordance with augmented MPS via mTORC1 is cardinal for muscle growth as these events result in a positive net protein balance. However, like many endogenous processes, including inflammation, prolonged activation often leads to detrimental effects and pathology. In the case of mTORC1, sustained activation via loss of TSC1 leads to muscle atrophy because in the absence of autophagy, an excess of damaged proteins and organelles accumulate, resulting in cell death12. In correspondence, exercise-induced autophagy has shown to complement skeletal muscle plasticity in response to training40. Beyond the previously described negative feedback loops and crosstalk interactions that protect against excessive and/or insufficient mTORC1 activation, a significant regulatory dynamic has been discovered between mature IGF-1s and pro-IGF-1s. Despite the ability of mature IGF-1 to inhibit MPB and autophagy via mTOR activation, pro-IGF-1s in mice activate autophagy/ lysosome systems64. It is proposed that this effect of pro-IGF-1s preserves skeletal muscle in aging due to the autophagic optimization of cellular functions64. In essence, these findings suggest that long-term muscle growth is best achieved by incorporating adequate, but calculated, intervals of autophagy into a preferentially anabolic environment.
In a study on the latency and duration of MPS stimulation in response to amino acid infusion, it was found that the elevated MPS response to leucine/protein ingestion returns to baseline approximately 2 hours after ingestion, despite elevated plasma leucine content and mTOR signaling (including mTOR targets, S6K, and 4E-BP1) persisting above baseline for 5 hours76,77. Thus, given that all other variables are optimized, it is not only a finite rate of nutrient absorption and assimilation that restricts the rate of MPS. Rather, a major limiting factor for muscle growth appears to be the temporary desensitization of skeletal muscle to the anabolic effects of food. Considering this refractory period, Norton and Wilson78 advise 4 to 6 hours in between each leucine/protein-rich meal to maximize daily MPS. However, in a period of 12 hours, healthy resistance trained men who consumed 20 g of protein every 3 hours demonstrated higher MPS area under the curve than groups who consumed either 40 g of protein every 6 hours or 10 g of protein every 1.5 hours79. Thus, the MPS refractory period and optimal frequency of meals may depend on training status and/or body composition. Indeed, daily micronutrient and macronutrient recommendations vary greatly based on factors such as physical activity level, sex, body mass, and goals. As a highly metabolic tissue, skeletal muscle possesses the ability to rapidly allocate and expend macronutrient substrates (i.e., glucose) and this process is accelerated with exercise22,68. Specifically, the insulin-sensitizing effects of exercise are well-established1. Hence, muscle-bound individuals may possess a more flexible mTOR/MPS system that can accommodate higher meal frequencies. Relevantly, for highly active individuals attempting to accrete as much muscle as possible, eating more frequently can facilitate consuming the massive amount of food needed to achieve a hypercaloric state, the most optimal energy balance for muscle growth80. Apart from food, many athletes incorporate supplements for performance-enhancing benefits. Intermittent supplementation of branched-chain amino acids and β-hydroxy-β-methyl butyrate aids in establishing a preferentially anabolic environment via mTOR activation70,81. Based on the evidence above, when considering the incorporation of mTOR-stimulating dietary supplements, our conjecture is to factor in the refractory nature of mTOR which may compromise maximal anabolic responses to real food.
Effective training is characterized by appropriate intensity, volume, exercise selection, and rest, leading to desired adaptations4. Based on our previously discussed topics, these adaptations are calibrated by inflammatory signals, satellite cells, nutrients, MPS, and MPB (i.e., autophagy)9,33,40,44,71,77. Resistance exercise stimulates both MPS and MPB but preferentially sensitizes skeletal muscles to the anabolic effects of protein consumption and subsequent hormonal responses, which results in an overall growth of protein tissue, especially when paired with a caloric surplus/high-protein diet82. To put it simply, the acute catabolic event of resistance exercise potentiates the subsequent activation of anabolic pathways. We refer to this phenomenon as the anabolic priming by resistance exercise effect. Many of the dynamics we have previously detailed in this review fit this criterion. For instance, protein/carbohydrate consumption shortly following an efficient exercise protocol maximizes the myotropic effects of the proteins/carbohydrates chiefly due to the interplay between AMPK and mTOR. High AMPK signaling during exercise abruptly inhibits mTOR68. Hence, it may be inferred that exercise truncates the mTOR refractory period, sensitizes the MPS system, and promotes a maximal anabolic response given an adequate post-workout meal. This is in line with the insulin-sensitizing effect of exercise which may ameliorate insulin resistance caused by protein intake as part of the negative feedback loop involving the insulin/IRS/mTOR axis1,11,12,71. In addition to AMPK signaling, skeletal muscle perfusion also demonstrates an anabolic priming effect. Blood functions as a medium to support cellular metabolism and contains numerous signaling molecules. As such, increased blood flow during exercise aids in transporting growth factors and nutrients to downstream effectors. Moreover, spatial priming and adaptive responses to cellular swelling corresponding sarcoplasmic hypertrophy further exemplify the muscle pump as an anabolic primer5,26,27. At the cell’s surface, an acute bout of resistance exercise has shown to upregulate IGF-1 receptor expression in type I muscle fibers, but not in type II muscle fibers83. However, resistance exercise has shown to upregulate glucose transporter 4 expression in both type I and type II muscle fibers83. Since the density of such receptors significantly dictate a cell’s responsiveness to stimuli, exercise-induced receptor modulation may also contribute to an anabolic priming effect, though these adaptations may be fiber specific and variable in nature.
Mechanotransduction is the conversion of mechanical stress into biochemical signals, yielding accelerated MPS and other anabolic responses62. The MAPK cascade composes a series of kinases that transduce extracellular signals (i.e., mechanical tension) into cellular responses (i.e., myofibrillar hypertrophy). To accomplish this wide-ranging goal, the MAPK cascade modulates gene expression, redox status, and metabolism as well as cellular programs such as proliferation and differentiation4,84. Activators of MAPK include growth factors, cytokines, stress, and transforming growth factor-β (TGF-β) members84. Three families of MAPK have been characterized in mammalian cells: (1) the classical MAPK (also known as ERK), (2) JNK/stress-activated protein kinase, and (3) p38 kinase84. Among these modules, JNK appears to be the most responsive to mechanical tension and muscle damage, as exercise-induced JNK activation leads to a rapid rise in the mRNA of transcription factors involved in cell proliferation4. Low levels of reactive oxygen species generated during muscle contractions can lead to mTOR activation through MAPK-dependent pathways25,64. High intensity/unaccustomed exercise has shown to activate all three modules (ERK1/2, JNK, and p38), whereas low intensity/accustomed exercise has shown to activate only ERK1/22. While ERK1/2 stimulates acute substrate metabolism (i.e., fatty acid oxidation), p38 MAPK and JNK are proposed to propagate transcription-dependent adaptations2. In other words, it appears that high intensity/unaccustomed exercise is necessary to elicit significant changes in gene and protein expression necessary for long-term adaptation. Thus, adequate intensity and variability in exercise programs serve a purpose. MAPKs are integrated into various checkpoints of skeletal muscle regeneration/growth following muscle damage, including upstream of the NF-κB network, as well as downstream of the noncanonical Wnt signaling pathway2,51. Crosstalk between MAPKs and NF-κB influences the expression of genes related to inflammation (i.e., prostaglandins and COX enzymes), vasodilation (i.e., nitric oxide synthase), and satellite cell activation (i.e., myocyte-enhancing factor 2 [MEF2], a transcription factor interacting with MRFs)2,18. It is also suggested that the MAPK/ERK cascade supports amino acid sensing and coregulates mTORC1-induced MPS by inhibiting TSC262. In turn, Akt negatively regulates ERK, and the MAPK/ERK and Akt/mTORC1/S6K axes converge to coregulate numerous cell survival, proliferation, metabolism, and motility genes85.
Skeletal muscle is highly plastic, meaning that it can accommodate a wide range of adaptations beyond the apparent changes in muscle size. For example, substrate-specific metabolic adaptions are attributed to the type of exercise performed (i.e., strength vs. endurance training)2,40. The corresponding modifications to mitochondrial biosynthesis/degradation and oxidative capacity of muscle cells appear to be governed through calcium signaling24. Physical activity, Wnt/Ca2+, IGF-1, and PGF2-α can increase intracellular calcium levels4,37,53,86. Downstream effectors of calcium include calmodulin, a calcium-binding messenger protein; calcineurin (CnA), a major calmodulin-binding protein that mediates MEF2 and the hypertrophic effects of IGF-14,87; CaMK; PKC, which interacts with MAPK to induce transcription85; and NFAT86. Both NFAT and MEF2 are myogenic transcription factors downstream of CnA and CaMK24. Oxidative muscle fibers maintain higher resting concentrations of intracellular Ca2+ and mitochondrial content than their glycolytic counterparts23. Appropriately, high-volume/endurance-focused training induces PGC-1α, CaMK, CnA, and AMPK signaling to augment mitochondrial biogenesis and consequently, one’s endurance/oxidative capacity73,88. Indeed, this is the preferred training approach when attempting to elicit type I muscle fiber-specific adaptions and sarcoplasmic hypertrophy. Conversely, heavy resistance training favors the stimulation of myofibrillar protein synthesis via the Akt/mTOR pathway, which is otherwise interrupted by high AMPK signaling28,68,88. This is in line with the inverse relationship between striated muscle fiber size and oxidative capacity since compared to type I muscle fibers, type II muscle fibers are better suited for myofibrillar hypertrophy stimulated by intense resistance training24,28. Moreover, these interactions explain the concurrent training effect, where simultaneous strength and endurance training compromises adaptations due to the interference of biochemical pathways88. Although the multiple calcium-dependent pathways appear to preferentially stimulate oxidative adaptations, CnA signaling has been linked to hypertrophy in all fiber types4,87. This may be due to the role of the CnA/NFAT pathway down-regulating myostatin, the primary obstacle to muscle growth86.
Potent negative regulators of muscle growth are highly conserved across species89. Specifically, skeletal muscle mass is tightly controlled by myostatin, also known as growth/differentiation factor (GDF)-8. Myostatin is a member of the TGF-β family of proteins, which mediate cell growth, proliferation, and differentiation90. In the canonical (Smad-dependent) myostatin signaling pathway, free myostatin activates activin type II receptors (ActRIIA/ActRIIB), which leads to the activation of type I activin receptors (ALK4/ALK5)91. ALK4/ALK5 then stimulates the formation of a Smad protein heterooligomer complex that transitions into the nucleus to induce the expression of target genes91. Endogenous negative regulators of myostatin signaling include myostatin pro-peptide, GDF-associated serum protein-1/2, Smad7, and follistatin92,93. The exact transcriptional targets of myostatin remain ill-defined, though it is suggested that transcriptional outputs can depend on the cell/tissue type and physiological context94. Potential interactions with noncanonical (MAPK-dependent) myostatin signaling adds further complexity to the roles of myostatin94. There appears to be an inverse relationship between the oxidative capacity of a muscle and the level of myostatin expression24. Hence, a muscle fiber-type size paradox emanates, part of which describes that despite the higher hypertrophic potential of type II muscle fibers, glycolytic fibers also express higher levels of myostatin compared to oxidative fibers24. Although this relationship raises several questions, it is in agreement with the fact that a higher proportion of type II muscle fibers are present in myostatin deficiency94. Additionally, there are conflicting findings on the effects of resistance training on myostatin expression. Some studies report that resistance exercise increases basal myostatin mRNA levels, while others report the very opposite91. Differences in exercise modality, sample demographics, and/or the timing of sample collection likely contribute to this discrepancy. Nonetheless, more research is needed to determine the specific role and expression pattern of myostatin in humans.
Myostatin is integrated into various muscle turnover pathways, including the myogenic stem cell lineage90,91. Satellite cell proliferation and differentiation are interrupted by myostatin signaling as shown by its ability to inhibit Akt, promote GSK3-β activation, and down-regulate PAX7 and MRF expression91. In turn, Akt inhibition leads to the upregulation of FOXO transcription factors, promoting proteolytic processes91. FOXO1 also increases myostatin expression, demonstrating a feed-forward loop between myostatin and FOXO transcription factors24. Despite these effects, it has been suggested that the primary purpose of myostatin is not to induce atrophy directly, but rather to block excessive hypertrophy95. Synthetic knock-out or naturally occurring mutations that delete the myostatin gene across various species have been shown to cause drastic increases in skeletal muscle mass. Myostatin-null animals have approximately twice the muscle mass of wild-type animals and this additional muscle mass appears to stem from both hyperplasia and hypertrophy89,96. Moreover, myostatin-null mice exhibit improved insulin sensitivity and reduced adiposity attributed to their increased muscle mass96. Although rare, organic mutations in the myostatin gene that result in significantly reduced myostatin production also occur in humans97. These people expectedly display a hyper-muscular phenotype. In myofibers, myostatin blockade can induce significant muscle growth in the absence of satellite cell activity and changes to the myonuclear domain90. Akt inhibition via myostatin deters mTOR-mediated MPS in skeletal muscle, thus, the anti-hypertrophic effects of myostatin extend beyond satellite cell inhibition91.
Since myostatin is mainly confined to skeletal muscle tissue, myostatin inhibition has emerged as a promising therapeutic approach for sarcopenia and other muscle wasting diseases96,98. Muscle mass of wild-type mice injected with a soluble myostatin decoy receptor, ActRIIB-Fc, increased by 40%–60% in only 2 weeks98. Myostatin-selective antibodies and follistatin-based therapies have also shown to cause drastic muscle growth and/or ameliorate muscle wasting98. Follistatin impedes multiple members of the TGF-β family, including myostatin, and follistatin transgene integration into myostatin-null mice causes a “quadruple muscle mass” effect89. These findings suggest TGF-β members apart from myostatin also exist to negatively regulate skeletal muscle mass and advocate myostatin inhibition in the clinical setting of muscle wasting. As the name suggests, transforming growth/growth differentiation factors influence the terminal differentiation of non-committed cells, sometimes diverting a cell toward an alternative lineage. In this respect, myostatin inhibition may not be as selective and desirable as previously perceived due to its influence on stem cells and tendon integrity. Pluripotent stem cells are directed toward specific lineages based on different cues. For instance, PAX7 denotes a stem cell’s commitment toward the myogenic lineage13. In a surgical research study, myostatin has shown the ability to divert the differentiation of rat bone marrow mesenchymal stem cells toward the tenocyte lineage99. Moreover, tendons of myostatin-deficient mice are 40% smaller and exhibit significant reductions in fibroblast density and type I collagen content compared to wild-type controls95. These findings can be explained by the fact that multiple collagen-related genes are upregulated by myostatin, proposedly via Smad-dependent mechanisms100. The apparent negative and positive effects that myostatin inhibition has on connective tissues and striated contractile tissues, respectively, may predispose one to injury and disease. Collagen is not only present at the muscle/bone interface (i.e., the distal/proximal tendon), but is also found ubiquitously as a supportive structure, including surrounding contractile muscle fibers (i.e., as fascicles) to protect against stretch-stress100. One reported side effect of soluble ActRIIB-Fc administration in humans is gum bleeding98. This symptom emulates scurvy, a disease characterized by a lack of vitamin C and subsequent deleterious changes to connective tissues. Moreover, in the previously described muscle fiber-type size paradox, type II muscle fibers paradoxically express higher levels of myostatin despite their greater cross-sectional area compared to type I muscle fibers24. Also, myostatin loss leads to a preferential increase in type II muscle fibers94. These discoveries suggest that the role of myostatin may be to synchronize tendon/connective tissue integrity and contractile protein mass by modifying multiple biochemical pathways and stem cell commitment (i.e., diverting stem cells away from the myogenic lineage and toward the tenocytic lineage). Indeed, glycolytic fibers can generate a higher peak force compared to oxidative fibers. A higher muscle fiber peak force necessitates a correspondingly higher tendon load capacity, providing a rationale for type II muscle fibers to express higher myostatin levels. Nutrient allocation and other biochemical pathways are also likely affected in a similar manner since the anti-myogenic effects of myostatin extend past satellite cell inhibition91. Beyond myostatin, additional TGF-β members are anticipated to modulate stem cell distribution and maintain physiologic proportions of tissue turnover.
In summary, the anabolic priming by resistance exercise effect describes how resistance exercise serves as an acute catabolic event to potentiate multiple anabolic pathways (Fig. 1). The initial inflammatory response following muscle damage is primarily mediated through NF-κB, prostaglandins, and COX enzymes2,36,41,46. Subsequently, satellite cells fuse to damaged myofibers as part of myofibrillar hypertrophy and this process is complemented by sarcoplasmic hypertrophy27. The sequence of the myogenic lineage is chiefly regulated by the Wnt signaling pathways and supported by pro-IGF-1 isoforms13. The MGF hypothesis elucidates unique roles of pro-IGF-1s preceding their cleavage into mature IGF-1, which in turn, emerges as a potent activator of the PI3K/Akt/mTOR and MPS64-66,70,75. Multiple pathways influence skeletal muscle plasticity, partially via interactions with calcium signaling24. Also, differential activation of the MAPK/ERK cascade emphasizes the importance of adequate training intensity and variability2. It is apparent that numerous crosstalk interactions and negative feedback loops govern muscle protein turnover. These dynamic interactions appear to maintain homeostasis and health/longevity, but also complicate the process of optimizing exercise and nutrition variables (i.e., exercise timing, frequency, and type; as well as macronutrient ratios). Although some literature may suggest objective values for these variables, we propose that it is most pertinent to continuously evaluate one’s progress and adjust variables accordingly due to vast individual differences in genetics, lifestyle, and goals. In the case one’s goal relates to bodybuilding, it is inevitable for one to face a plateau in muscle growth, as the threshold of anabolic stimulus needed for continuous adaptations rises beyond reach and the ability to generate a sufficient inflammatory response deteriorates. In addition, myostatin impedes excessive hypertrophy through multiple vectors90,91. To surpass natural limits of body composition and performance, some may experiment with performance-enhancing drugs (PEDs)10. Several hormones not detailed in this review, such as testosterone and estrogen, are also involved in skeletal muscle anabolism3,10. These sex steroids are the basis of anabolic-androgenic steroid (AAS) development and appear to govern long-term body composition beyond exercise and nutrition variables by manipulating many of the pathways outlined in the present review. To read more on the mechanisms of AAS and other PEDs, as well as the highly conserved homeostatic system of skeletal muscle regulation, we recommend the review, “The mechanisms of anabolic steroids, selective androgen receptor modulators and myostatin inhibitors” by Park et al.10, Clearly, investigating the science of exercise physiology reveals insightful findings on the finely tuned ability of the human body to adapt to stress. Future research is needed to expand off current literature and clarify discrepancies such as the pattern of myostatin expression in relation to resistance exercise. With great interest, we anticipate the discovery of new paradigms and novel regulators of skeletal muscle turnover.
REFERENCES
1. Ryan AS. 2010; Exercise in aging: its important role in mortality, obesity and insulin resistance. Aging Health. 6:551–63. DOI: 10.2217/ahe.10.46. PMID: 21359160. PMCID: PMC3042702.


2. Kramer HF, Goodyear LJ. 2007; Exercise, MAPK, and NF-kappaB signaling in skeletal muscle. J Appl Physiol (1985). 103:388–95. DOI: 10.1152/japplphysiol.00085.2007. PMID: 17303713.
3. Kraemer WJ, Ratamess NA. 2005; Hormonal responses and adaptations to resistance exercise and training. Sports Med. 35:339–61. DOI: 10.2165/00007256-200535040-00004. PMID: 15831061.


4. Schoenfeld BJ. 2010; The mechanisms of muscle hypertrophy and their application to resistance training. J Strength Cond Res. 24:2857–72. DOI: 10.1519/JSC.0b013e3181e840f3. PMID: 20847704.


5. Schoenfeld BJ, Contreras B. 2014; The muscle pump: potential mechanisms and applications for enhancing hypertrophic adaptations. Strength Cond J. 36:21–5. DOI: 10.1519/SSC.0000000000000021.
6. Yin H, Price F, Rudnicki MA. 2013; Satellite cells and the muscle stem cell niche. Physiol Rev. 93:23–67. DOI: 10.1152/physrev.00043.2011. PMID: 23303905. PMCID: PMC4073943.


7. Tesch PA. 1988; Skeletal muscle adaptations consequent to long-term heavy resistance exercise. Med Sci Sports Exerc. 20(5 Suppl):S132–4. DOI: 10.1249/00005768-198810001-00008. PMID: 3057312.


8. Schmidt M, Schüler SC, Hüttner SS, von Eyss B, von Maltzahn J. 2019; Adult stem cells at work: regenerating skeletal muscle. Cell Mol Life Sci. 76:2559–70. DOI: 10.1007/s00018-019-03093-6. PMID: 30976839. PMCID: PMC6586695.


9. Adams GR. 2006; Satellite cell proliferation and skeletal muscle hypertrophy. Appl Physiol Nutr Metab. 31:782–90. DOI: 10.1139/h06-053. PMID: 17213900.


10. Park J, Mcllvain V, Rosenberg J, Donovan L, Desai P, Kim JY. 2022; The mechanisms of anabolic steroids, selective androgen receptor modulators and myostatin inhibitors. Korean J Sports Med. 40:67–85. DOI: 10.5763/kjsm.2022.40.2.67.


11. Laplante M, Sabatini DM. 2012; mTOR signaling in growth control and disease. Cell. 149:274–93. DOI: 10.1016/j.cell.2012.03.017. PMID: 22500797. PMCID: PMC3331679.


12. Saxton RA, Sabatini DM. 2017; mTOR signaling in growth, metabolism, and disease. Cell. 168:960–76. DOI: 10.1016/j.cell.2017.02.004. PMID: 28283069. PMCID: PMC5394987.


13. Seale P, Asakura A, Rudnicki MA. 2001; The potential of muscle stem cells. Dev Cell. 1:333–42. DOI: 10.1016/S1534-5807(01)00049-1.


14. Relaix F, Zammit PS. 2012; Satellite cells are essential for skeletal muscle regeneration: the cell on the edge returns centre stage. Development. 139:2845–56. DOI: 10.1242/dev.069088. PMID: 22833472.
15. Brook MS, Wilkinson DJ, Smith K, Atherton PJ. 2019; It's not just about protein turnover: the role of ribosomal biogenesis and satellite cells in the regulation of skeletal muscle hypertrophy. Eur J Sport Sci. 19:952–63. DOI: 10.1080/17461391.2019.1569726. PMID: 30741116.


16. Murach KA, Englund DA, Dupont-Versteegden EE, McCarthy JJ, Peterson CA. 2018; Myonuclear domain flexibility challenges rigid assumptions on satellite cell contribution to skeletal muscle fiber hypertrophy. Front Physiol. 9:635. DOI: 10.3389/fphys.2018.00635. PMID: 29896117. PMCID: PMC5986879.


17. Bruusgaard JC, Johansen IB, Egner IM, Rana ZA, Gundersen K. 2010; Myonuclei acquired by overload exercise precede hypertrophy and are not lost on detraining. Proc Natl Acad Sci U S A. 107:15111–6. DOI: 10.1073/pnas.0913935107. PMID: 20713720. PMCID: PMC2930527.


18. Hernández-Hernández JM, García-González EG, Brun CE, Rudnicki MA. 2017; The myogenic regulatory factors, determinants of muscle development, cell identity and regeneration. Semin Cell Dev Biol. 72:10–8. DOI: 10.1016/j.semcdb.2017.11.010.


19. Sousa-Victor P, García-Prat L, Muñoz-Cánoves P. 2022; Control of satellite cell function in muscle regeneration and its disruption in ageing. Nat Rev Mol Cell Biol. 23:204–26. DOI: 10.1038/s41580-021-00421-2. PMID: 34663964.
20. Haun CT, Vann CG, Roberts BM, Vigotsky AD, Schoenfeld BJ, Roberts MD. 2019; A critical evaluation of the biological construct skeletal muscle hypertrophy: size matters but so does the measurement. Front Physiol. 10:247. DOI: 10.3389/fphys.2019.00247. PMID: 30930796. PMCID: PMC6423469.


21. Antonio J, Gonyea WJ. 1993; Skeletal muscle fiber hyperplasia. Med Sci Sports Exerc. 25:1333–45. DOI: 10.1249/00005768-199312000-00004. PMID: 8107539.


22. Haun CT, Vann CG, Osburn SC, et al. 2019; Muscle fiber hypertrophy in response to 6 weeks of high-volume resistance training in trained young men is largely attributed to sarcoplasmic hypertrophy. PLoS One. 14:e0215267. DOI: 10.1371/journal.pone.0215267. PMID: 31166954. PMCID: PMC6550381.


23. Picard M, Hepple RT, Burelle Y. 2012; Mitochondrial functional specialization in glycolytic and oxidative muscle fibers: tailoring the organelle for optimal function. Am J Physiol Cell Physiol. 302:C629–41. DOI: 10.1152/ajpcell.00368.2011. PMID: 22031602.


24. van Wessel T, de Haan A, van der Laarse WJ, Jaspers RT. 2010; The muscle fiber type-fiber size paradox: hypertrophy or oxidative metabolism? Eur J Appl Physiol. 110:665–94. DOI: 10.1007/s00421-010-1545-0. PMID: 20602111. PMCID: PMC2957584.


25. de Freitas MC, Gerosa-Neto J, Zanchi NE, Lira FS, Rossi FE. 2017; Role of metabolic stress for enhancing muscle adaptations: practical applications. World J Methodol. 7:46–54. DOI: 10.5662/wjm.v7.i2.46. PMID: 28706859. PMCID: PMC5489423.
26. Delp MD, Laughlin MH. 1998; Regulation of skeletal muscle perfusion during exercise. Acta Physiol Scand. 162:411–9. DOI: 10.1046/j.1365-201X.1998.0324e.x. PMID: 9578387.


27. Roberts MD, Haun CT, Vann CG, Osburn SC, Young KC. 2020; Sarcoplasmic hypertrophy in skeletal muscle: a scientific "Unicorn" or resistance training adaptation? Front Physiol. 11:816. DOI: 10.3389/fphys.2020.00816. PMID: 32760293. PMCID: PMC7372125.


28. Moore DR, Tang JE, Burd NA, Rerecich T, Tarnopolsky MA, Phillips SM. 2009; Differential stimulation of myofibrillar and sarcoplasmic protein synthesis with protein ingestion at rest and after resistance exercise. J Physiol. 587(Pt 4):897–904. DOI: 10.1113/jphysiol.2008.164087. PMID: 19124543. PMCID: PMC2669978.
29. Vann CG, Roberson PA, Osburn SC, et al. 2020; Skeletal muscle myofibrillar protein abundance is higher in resistance-trained men, and aging in the absence of training may have an opposite effect. Sports (Basel). 8:7. DOI: 10.3390/sports8010007. PMID: 31936810. PMCID: PMC7022975.


30. Douglas J, Pearson S, Ross A, McGuigan M. 2017; Eccentric exercise: physiological characteristics and acute responses. Sports Med. 47:663–75. DOI: 10.1007/s40279-016-0624-8. PMID: 27638040.


31. De Souza EO, Lowery RP, Wilson JM, et al. 2016; Effects of arachidonic acid supplementation on acute anabolic signaling and chronic functional performance and body composition adaptations. PLoS One. 11:e0155153. DOI: 10.1371/journal.pone.0155153. PMID: 27182886. PMCID: PMC4868363.


32. Schoenfeld BJ. 2012; The use of nonsteroidal anti-inflammatory drugs for exercise-induced muscle damage: implications for skeletal muscle development. Sports Med. 42:1017–28. DOI: 10.1007/BF03262309. PMID: 23013520.


33. Costamagna D, Costelli P, Sampaolesi M, Penna F. 2015; Role of inflammation in muscle homeostasis and myogenesis. Mediators Inflamm. 2015:805172. DOI: 10.1155/2015/805172. PMID: 26508819. PMCID: PMC4609834.
34. Arnold L, Henry A, Poron F, et al. 2007; Inflammatory monocytes recruited after skeletal muscle injury switch into antiinflammatory macrophages to support myogenesis. J Exp Med. 204:1057–69. DOI: 10.1084/jem.20070075. PMID: 17485518. PMCID: PMC2118577.


35. Markworth JF, Cameron-Smith D. 2013; Arachidonic acid supplementation enhances in vitro skeletal muscle cell growth via a COX-2-dependent pathway. Am J Physiol Cell Physiol. 304:C56–67. DOI: 10.1152/ajpcell.00038.2012. PMID: 23076795.


36. Veliça P, Bunce CM. 2008; Prostaglandins in muscle regeneration. J Muscle Res Cell Motil. 29:163–7. DOI: 10.1007/s10974-008-9154-9.


37. Korotkova M, Lundberg IE. 2014; The skeletal muscle arachidonic acid cascade in health and inflammatory disease. Nat Rev Rheumatol. 10:295–303. DOI: 10.1038/nrrheum.2014.2. PMID: 24468934.
38. Trappe TA, Liu SZ. 2013; Effects of prostaglandins and COX-inhibiting drugs on skeletal muscle adaptations to exercise. J Appl Physiol (1985). 115:909–19. DOI: 10.1152/japplphysiol.00061.2013. PMID: 23539318. PMCID: PMC3764617.


39. Palmer RM. 1990; Prostaglandins and the control of muscle protein synthesis and degradation. Prostaglandins Leukot Essent Fatty Acids. 39:95–104. DOI: 10.1016/0952-3278(90)90017-F.


40. Sanchez AM, Bernardi H, Py G, Candau RB. 2014; Autophagy is essential to support skeletal muscle plasticity in response to endurance exercise. Am J Physiol Regul Integr Comp Physiol. 307:R956–69. DOI: 10.1152/ajpregu.00187.2014. PMID: 25121614.


41. Otis JS, Burkholder TJ, Pavlath GK. 2005; Stretch-induced myoblast proliferation is dependent on the COX2 pathway. Exp Cell Res. 310:417–25. DOI: 10.1016/j.yexcr.2005.08.009. PMID: 16168411.


42. Hoxha M. 2019; Duchenne muscular dystrophy: focus on arachidonic acid metabolites. Biomed Pharmacother. 110:796–802. DOI: 10.1016/j.biopha.2018.12.034. PMID: 30554118.
43. Webster JM, Kempen LJ, Hardy RS, Langen RC. 2020; Inflammation and skeletal muscle wasting during cachexia. Front Physiol. 11:597675. DOI: 10.3389/fphys.2020.597675. PMID: 33329046. PMCID: PMC7710765.
44. Woods JA, Wilund KR, Martin SA, Kistler BM. 2012; Exercise, inflammation and aging. Aging Dis. 3:130–40.
45. Cheung K, Hume P, Maxwell L. 2003; Delayed onset muscle soreness: treatment strategies and performance factors. Sports Med. 33:145–64. DOI: 10.2165/00007256-200333020-00005. PMID: 12617692.
46. Straughn AR, Hindi SM, Xiong G, Kumar A. 2019; Canonical NF-κB signaling regulates satellite stem cell homeostasis and function during regenerative myogenesis. J Mol Cell Biol. 11:53–66. DOI: 10.1093/jmcb/mjy053. PMID: 30239789.


47. Vella L, Markworth JF, Peake JM, Snow RJ, Cameron-Smith D, Russell AP. 2014; Ibuprofen supplementation and its effects on NF-κB activation in skeletal muscle following resistance exercise. Physiol Rep. 2:e12172. DOI: 10.14814/phy2.12172. PMID: 25344476. PMCID: PMC4254097.
48. Li H, Malhotra S, Kumar A. 2008; Nuclear factor-kappa B signaling in skeletal muscle atrophy. J Mol Med (Berl). 86:1113–26. DOI: 10.1007/s00109-008-0373-8. PMID: 18574572. PMCID: PMC2597184.


49. Ma B, Hottiger MO. 2016; Crosstalk between Wnt/β-catenin and NF-κB signaling pathway during inflammation. Front Immunol. 7:378. DOI: 10.3389/fimmu.2016.00378. PMID: 27713747. PMCID: PMC5031610.


50. Komiya Y, Habas R. 2008; Wnt signal transduction pathways. Organogenesis. 4:68–75. DOI: 10.4161/org.4.2.5851. PMID: 19279717. PMCID: PMC2634250.


51. von Maltzahn J, Bentzinger CF, Rudnicki MA. 2011; Wnt7a-Fzd7 signalling directly activates the Akt/mTOR anabolic growth pathway in skeletal muscle. Nat Cell Biol. 14:186–91. DOI: 10.1038/ncb2404. PMID: 22179044. PMCID: PMC3271181.


52. Castilho RM, Squarize CH, Chodosh LA, Williams BO, Gutkind JS. 2009; mTOR mediates Wnt-induced epidermal stem cell exhaustion and aging. Cell Stem Cell. 5:279–89. DOI: 10.1016/j.stem.2009.06.017. PMID: 19733540. PMCID: PMC2939833.


53. Sethi JK, Vidal-Puig A. 2010; Wnt signalling and the control of cellular metabolism. Biochem J. 427:1–17. DOI: 10.1042/BJ20091866. PMID: 20226003. PMCID: PMC4301310.


54. von Maltzahn J, Chang NC, Bentzinger CF, Rudnicki MA. 2012; Wnt signaling in myogenesis. Trends Cell Biol. 22:602–9. DOI: 10.1016/j.tcb.2012.07.008. PMID: 22944199. PMCID: PMC3479319.


55. Bornens M. 2008; Organelle positioning and cell polarity. Nat Rev Mol Cell Biol. 9:874–86. DOI: 10.1038/nrm2524. PMID: 18946476.


56. Le Grand F, Jones AE, Seale V, Scimè A, Rudnicki MA. 2009; Wnt7a activates the planar cell polarity pathway to drive the symmetric expansion of satellite stem cells. Cell Stem Cell. 4:535–47. DOI: 10.1016/j.stem.2009.03.013. PMID: 19497282. PMCID: PMC2743383.


57. Huraskin D, Eiber N, Reichel M, et al. 2016; Wnt/β-catenin signaling via Axin2 is required for myogenesis and, together with YAP/Taz and Tead1, active in IIa/IIx muscle fibers. Development. 143:3128–42. DOI: 10.1242/dev.139907. PMID: 27578179.


58. Ramakrishnan AB, Cadigan KM. 2017; Wnt target genes and where to find them. F1000Res. 6:746. DOI: 10.12688/f1000research.11034.1. PMID: 28649368. PMCID: PMC5464219.


59. Tidball JG, Welc SS. 2015; Macrophage-derived IGF-1 is a potent coordinator of myogenesis and inflammation in regenerating muscle. Mol Ther. 23:1134–5. DOI: 10.1038/mt.2015.97. PMID: 26122828. PMCID: PMC4817792.


60. Philippou A, Maridaki M, Pneumaticos S, Koutsilieris M. 2014; The complexity of the IGF1 gene splicing, posttranslational modification and bioactivity. Mol Med. 20:202–14. DOI: 10.2119/molmed.2014.00011. PMID: 24637928. PMCID: PMC4022784.


61. Osher E, Macaulay VM. 2019; Therapeutic targeting of the IGF axis. Cells. 8:895. DOI: 10.3390/cells8080895. PMID: 31416218. PMCID: PMC6721736.


62. Pasiakos SM. 2012; Exercise and amino acid anabolic cell signaling and the regulation of skeletal muscle mass. Nutrients. 4:740–58. DOI: 10.3390/nu4070740. PMID: 22852061. PMCID: PMC3407992.


63. Brisson BK, Barton ER. 2013; New modulators for IGF-I activity within IGF-I processing products. Front Endocrinol (Lausanne). 4:42. DOI: 10.3389/fendo.2013.00042. PMID: 23543904. PMCID: PMC3608916.


64. Ascenzi F, Barberi L, Dobrowolny G, et al. 2019; Effects of IGF-1 isoforms on muscle growth and sarcopenia. Aging Cell. 18:e12954. DOI: 10.1111/acel.12954. PMID: 30953403. PMCID: PMC6516183.


65. Philippou A, Papageorgiou E, Bogdanis G, et al. 2009; Expression of IGF-1 isoforms after exercise-induced muscle damage in humans: characterization of the MGF E peptide actions in vitro. In Vivo. 23:567–75.
66. Matheny RW Jr, Nindl BC, Adamo ML. 2010; Minireview: Mechano-growth factor: a putative product of IGF-I gene expression involved in tissue repair and regeneration. Endocrinology. 151:865–75. DOI: 10.1210/en.2009-1217. PMID: 20130113. PMCID: PMC2840678.


67. Aboalola D, Han VK. 2017; Different effects of insulin-like growth factor-1 and insulin-like growth factor-2 on myogenic differentiation of human mesenchymal stem cells. Stem Cells Int. 2017:8286248. DOI: 10.1155/2017/8286248. PMID: 29387091. PMCID: PMC5745708.


68. Dreyer HC, Fujita S, Cadenas JG, Chinkes DL, Volpi E, Rasmussen BB. 2006; Resistance exercise increases AMPK activity and reduces 4E-BP1 phosphorylation and protein synthesis in human skeletal muscle. J Physiol. 576(Pt 2):613–24. DOI: 10.1113/jphysiol.2006.113175. PMID: 16873412. PMCID: PMC1890364.


69. Frost RA, Lang CH. 2007; Protein kinase B/Akt: a nexus of growth factor and cytokine signaling in determining muscle mass. J Appl Physiol (1985). 103:378–87. DOI: 10.1152/japplphysiol.00089.2007. PMID: 17332274.


70. Laplante M, Sabatini DM. 2009; mTOR signaling at a glance. J Cell Sci. 122(Pt 20):3589–94. DOI: 10.1242/jcs.051011. PMID: 19812304. PMCID: PMC2758797.


71. Tremblay F, Marette A. 2001; Amino acid and insulin signaling via the mTOR/p70 S6 kinase pathway: a negative feedback mechanism leading to insulin resistance in skeletal muscle cells. J Biol Chem. 276:38052–60. DOI: 10.1074/jbc.M106703200. PMID: 11498541.
72. White JP, Gao S, Puppa MJ, Sato S, Welle SL, Carson JA. 2013; Testosterone regulation of Akt/mTORC1/FoxO3a signaling in skeletal muscle. Mol Cell Endocrinol. 365:174–86. DOI: 10.1016/j.mce.2012.10.019. PMID: 23116773. PMCID: PMC3529800.


73. Kjøbsted R, Hingst JR, Fentz J, et al. 2018; AMPK in skeletal muscle function and metabolism. FASEB J. 32:1741–77. DOI: 10.1096/fj.201700442R. PMID: 29242278. PMCID: PMC5945561.


74. Zhang P, Liang X, Shan T, et al. 2015; mTOR is necessary for proper satellite cell activity and skeletal muscle regeneration. Biochem Biophys Res Commun. 463:102–8. DOI: 10.1016/j.bbrc.2015.05.032. PMID: 25998386. PMCID: PMC4484853.
75. Dibble CC, Cantley LC. 2015; Regulation of mTORC1 by PI3K signaling. Trends Cell Biol. 25:545–55. DOI: 10.1016/j.tcb.2015.06.002. PMID: 26159692. PMCID: PMC4734635.


76. Bohé J, Low JF, Wolfe RR, Rennie MJ. 2001; Latency and duration of stimulation of human muscle protein synthesis during continuous infusion of amino acids. J Physiol. 532(Pt 2):575–9. DOI: 10.1111/j.1469-7793.2001.0575f.x. PMID: 11306673. PMCID: PMC2278544.
77. Atherton PJ, Etheridge T, Watt PW, et al. 2010; Muscle full effect after oral protein: time-dependent concordance and discordance between human muscle protein synthesis and mTORC1 signaling. Am J Clin Nutr. 92:1080–8. DOI: 10.3945/ajcn.2010.29819. PMID: 20844073.


78. Norton LE, Wilson GJ. 2009; Optimal protein intake to maximize muscle protein synthesis: examinations of optimal meal protein intake and frequency for athletes. Agro Food Ind Hi Tech. 20:54–7.
79. Areta JL, Burke LM, Ross ML, et al. 2013; Timing and distribution of protein ingestion during prolonged recovery from resistance exercise alters myofibrillar protein synthesis. J Physiol. 591:2319–31. DOI: 10.1113/jphysiol.2012.244897. PMID: 23459753. PMCID: PMC3650697.


80. Slater GJ, Dieter BP, Marsh DJ, Helms ER, Shaw G, Iraki J. 2019; Is an energy surplus required to maximize skeletal muscle hypertrophy associated with resistance training. Front Nutr. 6:131. DOI: 10.3389/fnut.2019.00131. PMID: 31482093. PMCID: PMC6710320.


81. Zanchi NE, Gerlinger-Romero F, Guimarães-Ferreira L, et al. 2011; HMB supplementation: clinical and athletic performance-related effects and mechanisms of action. Amino Acids. 40:1015–25. DOI: 10.1007/s00726-010-0678-0. PMID: 20607321.


82. Hector AJ, Phillips SM. 2018; Protein recommendations for weight loss in elite athletes: a focus on body composition and performance. Int J Sport Nutr Exerc Metab. 28:170–7. DOI: 10.1123/ijsnem.2017-0273. PMID: 29182451.
83. Gallagher PM, Touchberry CD, Teson K, McCabe E, Tehel M, Wacker MJ. 2013; Effects of an acute bout of resistance exercise on fiber-type specific to GLUT4 and IGF-1R expression. Appl Physiol Nutr Metab. 38:581–6. DOI: 10.1139/apnm-2012-0301. PMID: 23668768.
84. Zhang W, Liu HT. 2002; MAPK signal pathways in the regulation of cell proliferation in mammalian cells. Cell Res. 12:9–18. DOI: 10.1038/sj.cr.7290105. PMID: 11942415.
85. Mendoza MC, Er EE, Blenis J. 2011; The Ras-ERK and PI3K-mTOR pathways: cross-talk and compensation. Trends Biochem Sci. 36:320–8. DOI: 10.1016/j.tibs.2011.03.006. PMID: 21531565. PMCID: PMC3112285.


86. Al-Shanti N, Stewart CE. 2009; Ca2+/calmodulin-dependent transcriptional pathways: potential mediators of skeletal muscle growth and development. Biol Rev Camb Philos Soc. 84:637–52. DOI: 10.1111/j.1469-185X.2009.00090.x. PMID: 19725819.
87. Naya FJ, Mercer B, Shelton J, Richardson JA, Williams RS, Olson EN. 2000; Stimulation of slow skeletal muscle fiber gene expression by calcineurin in vivo. J Biol Chem. 275:4545–8. DOI: 10.1074/jbc.275.7.4545. PMID: 10671477.


88. Methenitis S. 2018; A brief review on concurrent training: from laboratory to the field. Sports (Basel). 6:127. DOI: 10.3390/sports6040127. PMID: 30355976. PMCID: PMC6315763.


89. Lee SJ, Lee YS, Zimmers TA, et al. 2010; Regulation of muscle mass by follistatin and activins. Mol Endocrinol. 24:1998–2008. DOI: 10.1210/me.2010-0127. PMID: 20810712. PMCID: PMC2954636.


90. Lee SJ, Huynh TV, Lee YS, et al. 2012; Role of satellite cells versus myofibers in muscle hypertrophy induced by inhibition of the myostatin/activin signaling pathway. Proc Natl Acad Sci U S A. 109:E2353–60. DOI: 10.1073/pnas.1206410109. PMID: 22869749. PMCID: PMC3435227.


91. Rodriguez J, Vernus B, Chelh I, et al. 2014; Myostatin and the skeletal muscle atrophy and hypertrophy signaling pathways. Cell Mol Life Sci. 71:4361–71. DOI: 10.1007/s00018-014-1689-x. PMID: 25080109.


92. Lee YS, Lee SJ. 2013; Regulation of GDF-11 and myostatin activity by GASP-1 and GASP-2. Proc Natl Acad Sci U S A. 110:E3713–22. DOI: 10.1073/pnas.1309907110. PMID: 24019467. PMCID: PMC3785741.


93. Zhu X, Topouzis S, Liang LF, Stotish RL. 2004; Myostatin signaling through Smad2, Smad3 and Smad4 is regulated by the inhibitory Smad7 by a negative feedback mechanism. Cytokine. 26:262–72. DOI: 10.1016/j.cyto.2004.03.007. PMID: 15183844.


94. Walker RG, Poggioli T, Katsimpardi L, et al. 2016; Biochemistry and biology of GDF11 and myostatin: similarities, differences, and questions for future investigation. Circ Res. 118:1125–42. DOI: 10.1161/CIRCRESAHA.116.308391. PMID: 27034275. PMCID: PMC4818972.
95. Mendias CL, Lynch EB, Gumucio JP, et al. 2015; Changes in skeletal muscle and tendon structure and function following genetic inactivation of myostatin in rats. J Physiol. 593:2037–52. DOI: 10.1113/jphysiol.2014.287144. PMID: 25640143. PMCID: PMC4405758.


96. Guo T, Jou W, Chanturiya T, Portas J, Gavrilova O, McPherron AC. 2009; Myostatin inhibition in muscle, but not adipose tissue, decreases fat mass and improves insulin sensitivity. PLoS One. 4:e4937. DOI: 10.1371/journal.pone.0004937. PMID: 19295913. PMCID: PMC2654157.


97. Schuelke M, Wagner KR, Stolz LE, et al. 2004; Myostatin mutation associated with gross muscle hypertrophy in a child. N Engl J Med. 350:2682–8. DOI: 10.1056/NEJMoa040933. PMID: 15215484.


98. Suh J, Lee YS. 2020; Myostatin inhibitors: panacea or predicament for musculoskeletal disorders? J Bone Metab. 27:151–65. DOI: 10.11005/jbm.2020.27.3.151. PMID: 32911580. PMCID: PMC7571243.


99. Le W, Yao J. 2017; The effect of myostatin (GDF-8) on proliferation and tenocyte differentiation of rat bone marrow-derived mesenchymal stem cells. J Hand Surg Asian Pac Vol. 22:200–7. DOI: 10.1142/S0218810417500253. PMID: 28506172.
100. Welle S, Cardillo A, Zanche M, Tawil R. 2009; Skeletal muscle gene expression after myostatin knockout in mature mice. Physiol Genomics. 38:342–50. DOI: 10.1152/physiolgenomics.00054.2009. PMID: 19509079. PMCID: PMC3774565.


Fig. 1
The biochemical transduction of resistance exercise. Resistance exercise stimulates calcium mobilization, AMPK and Wnt signaling, and growth hormone release while also causing metabolic stress, mechanical tension, and muscle damage. Crosstalk between the Wnt, MAPK (particularly the JNK subpathway), and NF-κB pathways coordinates satellite cell activation and the subsequent myonuclear accretion. It is suggested that the retention of such myonuclei is responsible for the muscle memory phenomenon. The MAPK pathway also increases fatty acid oxidation to improve body composition. Growth hormone causes the release of multiple pro-IGF-1s, while mechanical tension preferentially upregulates the MGF isoform. Pro-IGF-1s exert their own independent effects, while generating E-peptides and mature IGF-1. IGF-1, insulin, nutrients/amino acids, and other growth factors can stimulate the Akt/mTOR pathway. mTOR induces MPS and blocks FOXO signaling to inhibit MPB. Food ingestion demarcates a critical early checkpoint in the postexercise recovery period and is responsible for providing adequate nutrients as well as a stimulus for insulin secretion. AMPK signaling during exercise activates autophagic processes necessary for health and longevity. As part of the anabolic priming by resistance exercise effect, AMPK also abruptly inhibits mTOR and enhances insulin sensitivity, hyper-sensitizing the cell to anabolic stimuli during the subsequent postexercise recovery period. Metabolic stress during exercise causes skeletal muscle perfusion, defined as a transient increase in muscle blood flow and thickening of the sarcoplasm and/or sarcolemma. Like the insulin/mTOR sensitizing effect of AMPK, increased muscle blood flow promotes nutrient uptake and primes downstream anabolism via spatial priming. The combination of myonuclear accretion, MPS, and spatial priming yields myofibrillar hypertrophy. This increase in contractile tissue mass, in addition to increased glycogen synthesis via Wnt inhibition of GSK3-β, enhances insulin sensitivity and optimizes nutrient partitioning in the long-term. Exercise-induced calcium mobilization activates calcium-dependent pathways. In turn, transcription factors, such as NFAT and MEF2, induce transcriptional activity. Calcium signaling also promotes oxidative adaptations. The combination of optimized nutrient partitioning (i.e., larger/more receptive glycogen stores), increased contractile tissue mass, and oxidative adaptations results in greater exercise capacity. Thus, performance improvements are demonstrated in resistance training. Continuous skeletal muscle anabolism is limited by myostatin and the depletion of AA. However, these factors also appear to be attributed to the maintenance of tendon integrity and the anti-inflammatory effects of exercise, respectively. In summary, several interrelated routes govern skeletal muscle adaptations following resistance exercise. Note: Some relationships and signaling pathways are not shown for the purpose of simplicity. AMPK: AMP-activated protein kinase, MAPK: mitogen-activated protein kinase, JNK: c-Jun N-terminal kinase, NF-κB: nuclear factor κ-light-chain-enhancer of activated B cells, IGF-1: insulin-like growth factor 1, MGF: mechano growth factor, mTOR: mammalian target of rapamycin, MPS: muscle protein synthesis, FOXO: forkhead box O, MPB: muscle protein breakdown, GSK3-β: glycogen synthase kinase 3-β, NFAT: nuclear factor of activated T cells, MEF2: myocyte-enhancing factor 2, AA: arachidonic acid.
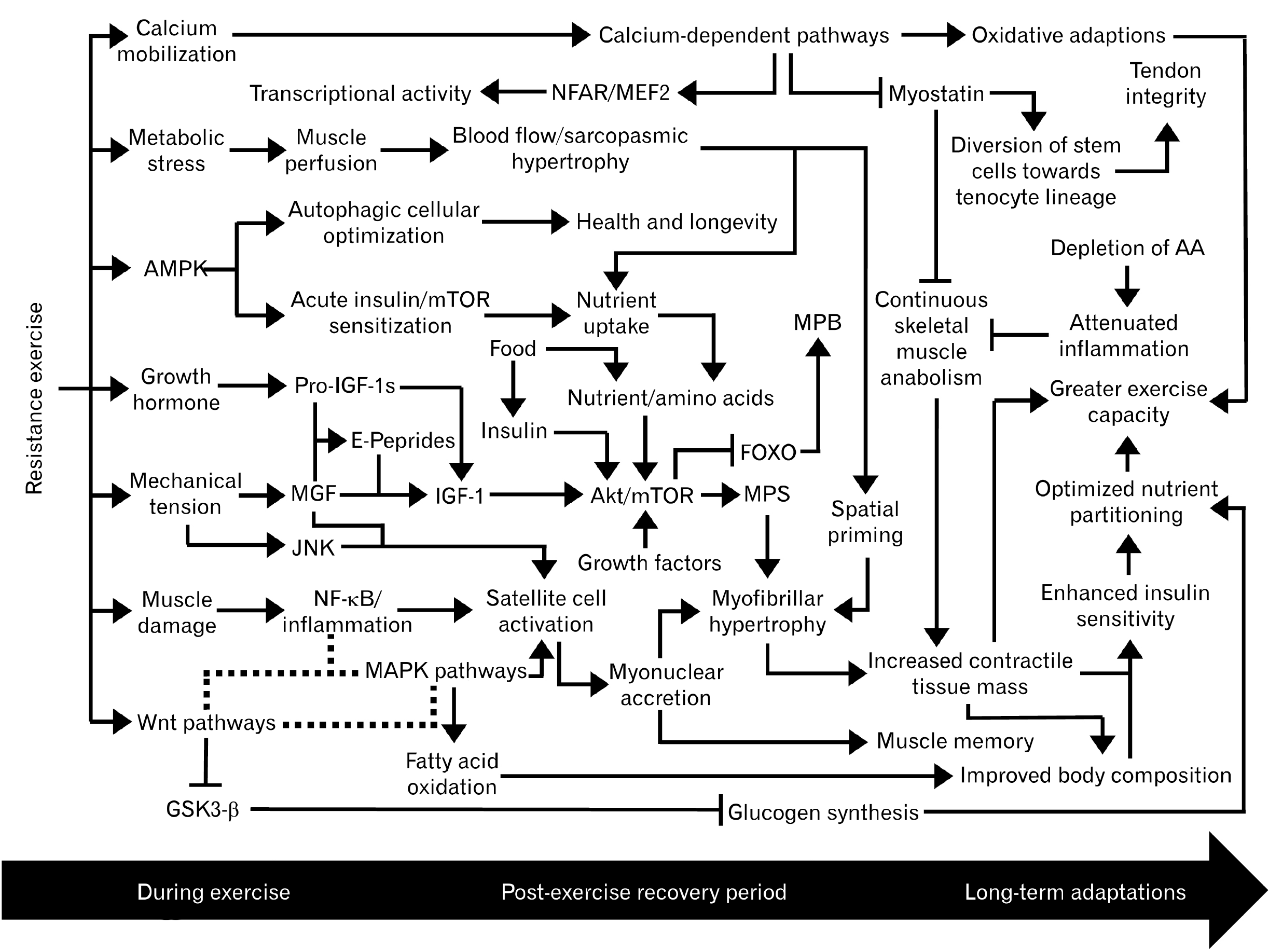