Abstract
It is well known that dopamine transmission from the ventral tegmental area (VTA) modulates motivated behavior and reinforcement learning. Although dopaminergic neurons are the major type of VTA neurons, recent studies show that a significant proportion of the VTA contains GABAergic and type 2 vesicular glutamate transporter (VGLUT2)-positive neurons. The non-dopaminergic neurons are also critically involved in regulating motivated behaviors. Some VTA neurons appear to co-release two different types of neurotransmitters. They are VGLUT2-DA neurons, VGLUT2-GABA neurons and GABA-DA neurons. These co-releasing neurons show distinct features compared to the neurons that release a single neurotransmitter. Here, we review how VTA cell populations wire to the other brain regions and how these projections differentially contribute to motivated behavior through the distinct molecular mechanism. We summarize the activities, projections and functions of VTA neurons concerning motivated behavior. This review article discriminates VTA cell populations related to the motivated behavior based on the neurotransmitters they release and extends the classical view of the dopamine-mediated reward system.
Dopamine transmissions from the midbrain have been characterized to mediate motivated behavior. In the mammalian brain, dopamine is released from ventral tegmental area (VTA) and substantia nigra (SN). Depending on the pre- and post-regions of dopamine, four distinct pathways exist: the mesolimbic, mesocortical, nigrostriatal, and tuberoinfundibular pathways (Fig. 1) [1-3]. The mesolimbic pathway represents dopamine traveling from the VTA to the nucleus accumbens (NAc). It encodes reward-associated memory and reinforcement learning [4]. The mesocortical pathway represents a dopaminergic projection from the VTA to the prefrontal cortex (PFC). This projection is related to cognition and executive behavior [5]. The nigrostriatal pathway is a dopaminergic projection from the SN to the striatum or basal ganglia, which mediates motor control [2,6]. Finally, the tuberoinfundibular pathway releases dopamine from the hypothalamus to the anterior pituitary [3].
Historically, the biological significance of dopamine in reward-seeking behavior has been controversial. There have been two theories regarding the role of dopamine. First, dopamine release reflects motivation [7]; specifically, Pavlovian motivation, as mice show increased movement to get the previously-encountered reward. Zhang et al. [8] first provided a computational model of this theory in 2009. Zhang et al. [8] defined motivation as the integration of the current state and associated cues during reward-associated training. Several studies have reported that the activity of dopamine neurons in the VTA and direct dopamine release at the axon terminal are correlated with motivated behaviors [9-11].
The second theory suggests that dopamine encodes reward prediction error (RPE). The RPE theory originated from value prediction after repetitive training. During the training, mice learn that they can get a positive stimulus, the unconditioned stimulus (US) when they encounter a specific cue, the conditioned stimulus (CS) [12,13]. This theory is supported by the dopamine dynamics in the brain region during exposure to CS and US. A rapid firing of dopaminergic neurons is observed upon exposing mice to an unexpected reward [14]. However, dopamine firing becomes below the basal level when anticipated stimulus is not given [15,16]. Furthermore, dopaminergic neurons show sharp firing to US during the initial learning. In well-trained animals, they often show a higher response to CS than US [17,18]. This finding supports that dopamine dynamics reflect the prediction error.
Whereas dopaminergic pathways and dopamine theories are well-known, non-dopaminergic neurons in the VTA have been underestimated. The contributions of non-dopaminergic, glutamatergic, or GABAergic neurons in the VTA have recently been emphasized regarding motivated behavior. In this article, we review distinct cell populations of the VTA that contribute to motivated behavior through projections and molecular pathways. Moreover, we suggest a role of neurons that release two neurotransmitters compared to that of neurons that release single neurotransmitters.
Dopamine neurons account for more than 70% of the total population of VTA neurons. These neurons activate when mice encounter positive stimuli such as food, sugar, water, or addictive drugs [19]. The two different firing patterns for dopamine neurons are phasic and tonic. Well-developed dopamine dynamics in the mammalian brain show that dopamine leaks to the post-region under basal conditions, whereas dopamine release increases after the stimulus [20]. Dopamine-releasing patterns, both phasic and tonic, can change the occupancy of the receptors in post-regions such as the NAc [21].
VTA dopaminergic neurons are highly connected to the ventral striatum [22]. In particular, the projection from the VTA dopaminergic neurons to the medium spiny neurons (MSNs) of the NAc is relevant to motivated behavior. MSNs can be divided into two populations: dopamine receptor subunit 1 expressing MSNs (D1-MSNs) and dopamine receptor subunit 2 expressing MSNs (D2-MSNs) [23]. From the classical perspective, the two different MSN subpopulations have distinct roles [24-26]. D1-MSNs encode reward/positive stimuli and D2-MSNs convey aversive/negative responses [27]. However, both types of MSNs function concurrently in reward-associated behavior [28].
When mice encounter a positive or aversive stimuli, dopamine is released from the axon terminals of the VTA dopaminergic neurons to the dendrites of NAc MSNs [29]. Two types of MSNs respond differentially according to the concentration of dopamine and have contrasting downstream signaling pathways. They display different projections as well [21]. For a classical understanding of projections from D1- and D2-MSNs, D1-MSNs directly inhibit the ventral mesencephalon (VM), which inhibits the thalamus (direct pathway). However, D2-MSNs disinhibit the VM by suppressing the ventral pallidum (VP) (indirect pathway) [27,30]. Distinct pathways have been upended by the finding that D1-MSNs convey an indirect pathway and that D2-MSNs pass through a direct pathway [30]. Based on this novel finding, the optogenetic activation of D1- and D2-MSNs respectively modulate self-stimulation, although the extent differs in each case [31].
Distinct populations of MSNs exhibit different intracellular calcium signaling cascades in response to dopamine [32]. After transient dopamine exposure, inositol 1,4,5-triphosphate (IP3)-mediated calcium signals are activated by D1 receptors (D1Rs), whereas IP3-evoked calcium signals are suppressed by D2 receptors (D2Rs) [33]. To summarize, intracellular calcium activity is enhanced in D1-MSNs but decreased in D2-MSNs, in response to dopamine. This calcium signaling pathway varies according to the concentration of dopamine [32,34]. When MSNs are exposed to high concentrations of dopamine, D1R-MSNs are active through enhancement of the PKA pathway, while D2-MSNs are inactive. On the other hand, once dopamine concentration is low, D2-MSNs become active through adenosine A2A receptor (A2AR) activation, which increases intracellular calcium levels [34].
Recent research suggests that VTA glutamatergic neurons also modulate motivated behavior [35,36]. Approximately 30% of VTA neurons are type 2 vesicular glutamate transporter (VGLUT2)-positive neurons [37,38]. They are specifically localized in the medial section of the VTA, such as the parabrachial pigmented nucleus and the interfascicular nucleus. Interestingly, the current study has shown that optogenetic activation of VTA glutamatergic neurons is sufficient to induce reward reinforcement behaviors [39]. Conditional knockout of VTA glutamatergic neurons causes reduced motivated behavior. Moreover, the fact that VTA VGLUT2 neurons become active during self-administration, drug-seeking behaviors, and reinforcement has been discovered [39-41]. VGLUT2-positive neurons in the VTA project to the NAc medial shell, VP, and lateral habenula (LHb). VTA VGLUT2-positive neurons excite NAc and VP neurons directly, whereas they suppress LHb neurons [42]. Once glutamate is released in the axon terminal, it binds to N-methyl-D-aspartate receptors (NMDA-Rs), and leads to calcium influx, which activates the pERK signaling pathway [43].
VTA VGLUT2 neurons are differentiated into two types: the VGLUT2-only-expressing neurons (VGLUT only neurons) and both VGLUT2 and dopamine-expressing neurons (VGLUT2-DA neurons) [44]. VGLUT2-DA neurons co-release glutamate and dopamine at the axon terminals. These neurons release glutamate through asymmetric synapses and dopamine through symmetric synapses. This new finding is contradictory to the classical viewpoint of Dale’s principle, which states that a single neuron releases a single type of neurotransmitter. VGLUT2-DA neurons increase the survivability and axonal arborization of VTA dopamine neurons [45,46]. In addition, this population projects to the cholinergic neurons in the NAc medial shell, and contributes to the switching of behavioral response during cued reinforcement tasks [38,45]. However, the studies regarding VGLUT2-DA neurons cannot suggest how these neurons act differentially from VGLUT2-only or DA-only neurons.
VTA VGLUT2 neurons also co-release the inhibitory neurotransmitter, GABA [47]. VGLUT2-GABA neurons are distinct from those of VGLUT-only neurons at the circuit level. VGLUT2-GABA neurons are highly connected to the LHb, whereas most VTA VGLUT2 neurons project to the NAc shell [42,48]. These two populations exhibit different activities for specific behaviors. In classical conditioning, VGLUT2-GABA neurons become active during exposure to a reward or electric shock. VGLUT2 only neurons are also active in response to the reward and electric shock. However, only this population shows active firing in response to the conditioned stimulus associated with the reward (CS+) [48]. To summarize, VGLUT2-GABA neurons encode the valence itself.
The GABAergic neurons form the largest population of VTA accounting for approximately 30% of the total VTA neuronal population [49]. Efferents of VTA GABAergic neurons project to the NAc, PFC, central amygdala, LHb, and dorsal raphe nucleus (DRN). The molecular functions of GABAergic synapses are distinct according to the type of GABA receptors. GABAA receptors cause hyperpolarization of post-neurons by the influx of chloride ions [50]. In contrast, GABAB receptors also induce hyperpolarization by suppressing acetyl cyclase and voltage-gated calcium channels (VGCC) [51].
GABAergic neurons have two distinct roles: inhibition of dopaminergic neurons in the VTA and inhibition of distal brain regions [49]. The function of VTA GABAergic neurons is quite complicated. Previous findings on the role of VTA GABAergic neurons have focused on the aversion and interruption of reward [52,53]. These experimental results imply that the activation of VTA GABAergic neurons activates aversion behavior and inactivates reward-consuming behavior [49]. However, VTA GABAergic neurons play a significant role in cue responsiveness and reward-associated learning [53,54]. VTA GABAergic neurons become active and suppress VTA DA neurons when mice expect a reward, such as sucrose or cocaine after they are exposed to a stimulus that is not associated with the reward [48,55,56]. This means that VTA GABAergic neurons interrupt reward consumption and disruption of responsiveness by gating dopaminergic activity in the VTA [57]. Moreover, mice show specific place preference, if the projection from caudal VTA GABAergic neurons to DRN serotonergic neurons is activated [58].
GABAergic neurons in the VTA also co-release dopamine or glutamate (GABA-DA neurons or VGLUT2-GABA neurons). GABA-dopamine co-releasing neurons highly target the NAc, whereas VGLUT2-GABA neurons project to the LHb and VP [49]. Interestingly, neurotransmitter release from these neurons is mediated by the uptake mechanism instead of the classical GAD1/2 pathway [59]. VTA DA neurons synthesize GABA through aldehyde dehydrogenase 1A1 [60]. GABA vesicle loading is mediated by a vesicular monoamine transporter instead of the vesicular GABA transporter (VGAT) [61]. However, the environmental stimuli that can activate GABA-DA neurons remain unknown.
This review summarizes that distinct populations of the VTA, which participate in motivated behavior by extending the classical view of the dopamine-mediated reward system. There are three different cell types, dopaminergic, glutamatergic, and GABAergic neuronal populations in the VTA. Each population affects motivated behavior through distinct mechanisms. Dopaminergic neurons project to the MSNs in the NAc. Released dopamine causes opposite downstream signaling, according to the type of dopaminergic receptors expressed in MSNs. VTA glutamatergic neurons also project to MSNs of the NAc shell and encode a reward-predicting cue (CS+). VTA GABAergic neurons mediate motivated behavior by suppressing VTA dopaminergic neurons or modulating distal regions, such as the LHb and DRN.
However, these three populations are not distinct. VGLUT2-DA, GABA-DA, and VGLUT2-GABA neurons exist, and their functions compared to those of single-expressing neurons are unclear. In accordance with current studies on the function of VTA neurons, they play a central role in motivated behavior as well as aversive experience [62-64]. Further research is required to understand how the functions of VTA neurons that release two other neurotransmitters are different from those of single-expressing neurons.
REFERENCES
1. Quessy F, Bittar T, Blanchette LJ, Lévesque M, Labonté B. 2021; Stress-induced alterations of mesocortical and mesolimbic dopaminergic pathways. Sci Rep. 11:11000. DOI: 10.1038/s41598-021-90521-y. PMID: 34040100. PMCID: PMC8154906. PMID: 25951ee047ed4c5a885a1dc34c91cdb1.


2. Bourdy R, Sánchez-Catalán MJ, Kaufling J, Balcita-Pedicino JJ, Freund-Mercier MJ, Veinante P, Sesack SR, Georges F, Barrot M. 2014; Control of the nigrostriatal dopamine neuron activity and motor function by the tail of the ventral tegmental area. Neuropsychopharmacology. 39:2788–2798. DOI: 10.1038/npp.2014.129. PMID: 24896615. PMCID: PMC4200489.


3. Stagkourakis S, Dunevall J, Taleat Z, Ewing AG, Broberger C. 2019; Dopamine release dynamics in the tuberoinfundibular dopamine system. J Neurosci. 39:4009–4022. DOI: 10.1523/JNEUROSCI.2339-18.2019. PMID: 30782976. PMCID: PMC6529860.


4. Spanagel R, Weiss F. 1999; The dopamine hypothesis of reward: past and current status. Trends Neurosci. 22:521–527. DOI: 10.1016/S0166-2236(99)01447-2. PMID: 10529820.


5. Naneix F, Marchand AR, Di Scala G, Pape JR, Coutureau E. 2012; Parallel maturation of goal-directed behavior and dopaminergic systems during adolescence. J Neurosci. 32:16223–16232. DOI: 10.1523/JNEUROSCI.3080-12.2012. PMID: 23152606. PMCID: PMC6794026.
6. Park J, Lim CS, Seo H, Park CA, Zhuo M, Kaang BK, Lee K. 2015; Pain perception in acute model mice of Parkinson's disease induced by 1-methyl-4-phenyl-1,2,3,6-tetrahydropyridine (MPTP). Mol Pain. 11:28. DOI: 10.1186/s12990-015-0026-1. PMID: 25981600. PMCID: PMC4448854.
7. Heinz A, Schlagenhauf F. 2010; Dopaminergic dysfunction in schizophrenia: salience attribution revisited. Schizophr Bull. 36:472–485. DOI: 10.1093/schbul/sbq031. PMID: 20453041. PMCID: PMC2879696.


8. Zhang JC, Lau PM, Bi GQ. 2009; Gain in sensitivity and loss in temporal contrast of STDP by dopaminergic modulation at hippocampal synapses. Proc Natl Acad Sci U S A. 106:13028–13033. DOI: 10.1073/pnas.0900546106. PMID: 19620735. PMCID: PMC2713390.


9. Schultz W, Apicella P, Ljungberg T. 1993; Responses of monkey dopamine neurons to reward and conditioned stimuli during successive steps of learning a delayed response task. J Neurosci. 13:900–913. DOI: 10.1523/JNEUROSCI.13-03-00900.1993. PMID: 8441015. PMCID: PMC6576600.


10. Aosaki T, Graybiel AM, Kimura M. 1994; Effect of the nigrostriatal dopamine system on acquired neural responses in the striatum of behaving monkeys. Science. 265:412–415. DOI: 10.1126/science.8023166. PMID: 8023166.


11. Mirenowicz J, Schultz W. 1996; Preferential activation of midbrain dopamine neurons by appetitive rather than aversive stimuli. Nature. 379:449–451. DOI: 10.1038/379449a0. PMID: 8559249.


12. Glimcher PW. 2011; Understanding dopamine and reinforcement learning: the dopamine reward prediction error hypothesis. Proc Natl Acad Sci U S A. 108(Suppl 3):15647–15654. Erratum in: Proc Natl Acad Sci U S A. 2011;108:17568-17569. DOI: 10.1073/pnas.1014269108. PMID: 21389268. PMCID: PMC3176615.


13. Morita K, Kato A. 2014; Striatal dopamine ramping may indicate flexible reinforcement learning with forgetting in the cortico-basal ganglia circuits. Front Neural Circuits. 8:36. Erratum in: Front Neural Circuits. 2014;8:48. DOI: 10.3389/fncir.2014.00036. PMID: 24782717. PMCID: PMC3988379.


14. Mohebi A, Pettibone JR, Hamid AA, Wong JT, Vinson LT, Patriarchi T, Tian L, Kennedy RT, Berke JD. 2019; Dissociable dopamine dynamics for learning and motivation. Nature. 570:65–70. Erratum in: Nature. 2019;571:E3. DOI: 10.1038/s41586-019-1235-y. PMID: 31118513. PMCID: PMC6555489.


15. Bromberg-Martin ES, Matsumoto M, Hikosaka O. 2010; Dopamine in motivational control: rewarding, aversive, and alerting. Neuron. 68:815–834. DOI: 10.1016/j.neuron.2010.11.022. PMID: 21144997. PMCID: PMC3032992.


16. Schultz W. 1998; Predictive reward signal of dopamine neurons. J Neurophysiol. 80:1–27. DOI: 10.1152/jn.1998.80.1.1. PMID: 9658025.
17. Bayer HM, Glimcher PW. 2005; Midbrain dopamine neurons encode a quantitative reward prediction error signal. Neuron. 47:129–141. DOI: 10.1016/j.neuron.2005.05.020. PMID: 15996553. PMCID: PMC1564381.


18. Cohen JY, Haesler S, Vong L, Lowell BB, Uchida N. 2012; Neuron-type-specific signals for reward and punishment in the ventral tegmental area. Nature. 482:85–88. DOI: 10.1038/nature10754. PMID: 22258508. PMCID: PMC3271183.


19. Matsumoto M, Hikosaka O. 2009; Two types of dopamine neuron distinctly convey positive and negative motivational signals. Nature. 459:837–841. DOI: 10.1038/nature08028. PMID: 19448610. PMCID: PMC2739096.


20. Grace AA. 1991; Phasic versus tonic dopamine release and the modulation of dopamine system responsivity: a hypothesis for the etiology of schizophrenia. Neuroscience. 41:1–24. DOI: 10.1016/0306-4522(91)90196-U. PMID: 1676137.


21. Dreyer JK, Herrik KF, Berg RW, Hounsgaard JD. 2010; Influence of phasic and tonic dopamine release on receptor activation. J Neurosci. 30:14273–14283. DOI: 10.1523/JNEUROSCI.1894-10.2010. PMID: 20962248. PMCID: PMC6634758.


22. Beier KT, Steinberg EE, DeLoach KE, Xie S, Miyamichi K, Schwarz L, Gao XJ, Kremer EJ, Malenka RC, Luo L. 2015; Circuit architecture of VTA dopamine neurons revealed by systematic input-output mapping. Cell. 162:622–634. DOI: 10.1016/j.cell.2015.07.015. PMID: 26232228. PMCID: PMC4522312.


23. Soares-Cunha C, de Vasconcelos NAP, Coimbra B, Domingues AV, Silva JM, Loureiro-Campos E, Gaspar R, Sotiropoulos I, Sousa N, Rodrigues AJ. 2020; Nucleus accumbens medium spiny neurons subtypes signal both reward and aversion. Mol Psychiatry. 25:3241–3255. Erratum in: Mol Psychiatry. 2020;25:3448. DOI: 10.1038/s41380-019-0484-3. PMID: 31462765. PMCID: PMC7714688.


24. Kwak S, Jung MW. 2019; Distinct roles of striatal direct and indirect pathways in value-based decision making. Elife. 8:e46050. DOI: 10.7554/eLife.46050. PMID: 31310237. PMCID: PMC6658164. PMID: 6460bd48f429464db7069fc206048432.


25. Sato D, Narita M, Hamada Y, Mori T, Tanaka K, Tamura H, Yamanaka A, Matsui R, Watanabe D, Suda Y, Senba E, Watanabe M, Navratilova E, Porreca F, Kuzumaki N, Narita M. 2022; Relief of neuropathic pain by cell-specific manipulation of nucleus accumbens dopamine D1- and D2-receptor-expressing neurons. Mol Brain. 15:10. DOI: 10.1186/s13041-021-00896-2. PMID: 34991655. PMCID: PMC8740378. PMID: 19e7e40f2ad442088b88dd6d70e75c50.


26. Cole SL, Chandra R, Harris M, Patel I, Wang T, Kim H, Jensen L, Russo SJ, Turecki G, Gancarz-Kausch AM, Dietz DM, Lobo MK. 2021; Cocaine-induced neuron subtype mitochondrial dynamics through Egr3 transcriptional regulation. Mol Brain. 14:101. DOI: 10.1186/s13041-021-00800-y. PMID: 34187517. PMCID: PMC8240292. PMID: 9d6dd2987a8d4db7bdc5e26bc0388cab.


27. Flanigan M, LeClair K. 2017; Shared motivational functions of ventral striatum D1 and D2 medium spiny neurons. J Neurosci. 37:6177–6179. DOI: 10.1523/JNEUROSCI.0882-17.2017. PMID: 28659329. PMCID: PMC5490058.


28. Kupchik YM, Brown RM, Heinsbroek JA, Lobo MK, Schwartz DJ, Kalivas PW. 2015; Coding the direct/indirect pathways by D1 and D2 receptors is not valid for accumbens projections. Nat Neurosci. 18:1230–1232. DOI: 10.1038/nn.4068. PMID: 26214370. PMCID: PMC4551610.


29. Gentry RN, Schuweiler DR, Roesch MR. 2019; Dopamine signals related to appetitive and aversive events in paradigms that manipulate reward and avoidability. Brain Res. 1713:80–90. DOI: 10.1016/j.brainres.2018.10.008. PMID: 30300635. PMCID: PMC6826219.


30. Kupchik YM, Kalivas PW. 2017; The direct and indirect pathways of the nucleus accumbens are not what you think. Neuropsychopharmacology. 42:369–370. DOI: 10.1038/npp.2016.160. PMID: 27909323. PMCID: PMC5143491.


31. Cole SL, Robinson MJF, Berridge KC. 2018; Optogenetic self-stimulation in the nucleus accumbens: D1 reward versus D2 ambivalence. PLoS One. 13:e0207694. DOI: 10.1371/journal.pone.0207694. PMID: 30496206. PMCID: PMC6264872. PMID: 989b2923ebc9433abd1eeb8721d9327b.
32. Swapna I, Bondy B, Morikawa H. 2016; Differential dopamine regulation of Ca2+ signaling and its timing dependence in the nucleus accumbens. Cell Rep. 15:563–573. DOI: 10.1016/j.celrep.2016.03.055. PMID: 27068462. PMCID: PMC4838497.


33. Nishi A, Kuroiwa M, Shuto T. 2011; Mechanisms for the modulation of dopamine D1 receptor signaling in striatal neurons. Front Neuroanat. 5:43. DOI: 10.3389/fnana.2011.00043. PMID: 21811441. PMCID: PMC3140648.


34. Zhang X, Nagai T, Ahammad RU, Kuroda K, Nakamuta S, Nakano T, Yukinawa N, Funahashi Y, Yamahashi Y, Amano M, Yoshimoto J, Yamada K, Kaibuchi K. 2019; Balance between dopamine and adenosine signals regulates the PKA/Rap1 pathway in striatal medium spiny neurons. Neurochem Int. 122:8–18. DOI: 10.1016/j.neuint.2018.10.008. PMID: 30336179.


35. Pupe S, Wallén-Mackenzie Å. 2015; Cre-driven optogenetics in the heterogeneous genetic panorama of the VTA. Trends Neurosci. 38:375–386. DOI: 10.1016/j.tins.2015.04.005. PMID: 25962754.


36. Bariselli S, Tzanoulinou S, Glangetas C, Prévost-Solié C, Pucci L, Viguié J, Bezzi P, O'Connor EC, Georges F, Lüscher C, Bellone C. 2016; SHANK3 controls maturation of social reward circuits in the VTA. Nat Neurosci. 19:926–934. DOI: 10.1038/nn.4319. PMID: 27273769. PMCID: PMC4948673.


37. Poulin JF, Caronia G, Hofer C, Cui Q, Helm B, Ramakrishnan C, Chan CS, Dombeck DA, Deisseroth K, Awatramani R. 2018; Mapping projections of molecularly defined dopamine neuron subtypes using intersectional genetic approaches. Nat Neurosci. 21:1260–1271. DOI: 10.1038/s41593-018-0203-4. PMID: 30104732. PMCID: PMC6342021.


38. Mingote S, Amsellem A, Kempf A, Rayport S, Chuhma N. 2019; Dopamine-glutamate neuron projections to the nucleus accumbens medial shell and behavioral switching. Neurochem Int. 129:104482. DOI: 10.1016/j.neuint.2019.104482. PMID: 31170424. PMCID: PMC6855309.


39. Zell V, Steinkellner T, Hollon NG, Warlow SM, Souter E, Faget L, Hunker AC, Jin X, Zweifel LS, Hnasko TS. 2020; VTA glutamate neuron activity drives positive reinforcement absent dopamine co-release. Neuron. 107:864–873.e4. DOI: 10.1016/j.neuron.2020.06.011. PMID: 32610039. PMCID: PMC7780844.


40. Birgner C, Nordenankar K, Lundblad M, Mendez JA, Smith C, le Grevès M, Galter D, Olson L, Fredriksson A, Trudeau LE, Kullander K, Wallén-Mackenzie A. 2010; VGLUT2 in dopamine neurons is required for psychostimulant-induced behavioral activation. Proc Natl Acad Sci U S A. 107:389–394. DOI: 10.1073/pnas.0910986107. PMID: 20018672. PMCID: PMC2806710.


41. Alsiö J, Nordenankar K, Arvidsson E, Birgner C, Mahmoudi S, Halbout B, Smith C, Fortin GM, Olson L, Descarries L, Trudeau LÉ, Kullander K, Lévesque D, Wallén-Mackenzie A. 2011; Enhanced sucrose and cocaine self-administration and cue-induced drug seeking after loss of VGLUT2 in midbrain dopamine neurons in mice. J Neurosci. 31:12593–12603. DOI: 10.1523/JNEUROSCI.2397-11.2011. PMID: 21880920. PMCID: PMC6703278.


42. Yoo JH, Zell V, Gutierrez-Reed N, Wu J, Ressler R, Shenasa MA, Johnson AB, Fife KH, Faget L, Hnasko TS. 2016; Ventral tegmental area glutamate neurons co-release GABA and promote positive reinforcement. Nat Commun. 7:13697. DOI: 10.1038/ncomms13697. PMID: 27976722. PMCID: PMC5171775. PMID: ebc4106e19444eaba72aa7e6cdab8f51.


43. Salery M, Trifilieff P, Caboche J, Vanhoutte P. 2020; From signaling molecules to circuits and behaviors: cell-type-specific adaptations to psychostimulant exposure in the striatum. Biol Psychiatry. 87:944–953. DOI: 10.1016/j.biopsych.2019.11.001. PMID: 31928716.


44. Hnasko TS, Hjelmstad GO, Fields HL, Edwards RH. 2012; Ventral tegmental area glutamate neurons: electrophysiological properties and projections. J Neurosci. 32:15076–15085. DOI: 10.1523/JNEUROSCI.3128-12.2012. PMID: 23100428. PMCID: PMC3685320.


45. Kouwenhoven WM, Fortin G, Penttinen AM, Florence C, Delignat-Lavaud B, Bourque MJ, Trimbuch T, Luppi MP, Salvail-Lacoste A, Legault P, Poulin JF, Rosenmund C, Awatramani R, Trudeau LÉ. 2020; VGluT2 expression in dopamine neurons contributes to postlesional striatal reinnervation. J Neurosci. 40:8262–8275. DOI: 10.1523/JNEUROSCI.0823-20.2020. PMID: 32928885. PMCID: PMC7577590.


46. Fortin GM, Bourque MJ, Mendez JA, Leo D, Nordenankar K, Birgner C, Arvidsson E, Rymar VV, Bérubé-Carrière N, Claveau AM, Descarries L, Sadikot AF, Wallén-Mackenzie Å, Trudeau LÉ. 2012; Glutamate corelease promotes growth and survival of midbrain dopamine neurons. J Neurosci. 32:17477–17491. DOI: 10.1523/JNEUROSCI.1939-12.2012. PMID: 23197738. PMCID: PMC6621856.


47. Miranda-Barrientos J, Chambers I, Mongia S, Liu B, Wang HL, Mateo-Semidey GE, Margolis EB, Zhang S, Morales M. 2021; Ventral tegmental area GABA, glutamate, and glutamate-GABA neurons are heterogeneous in their electrophysiological and pharmacological properties. Eur J Neurosci. 54:4061–4084. DOI: 10.1111/ejn.15156. PMID: 33619763. PMCID: PMC8380271.


48. Root DH, Barker DJ, Estrin DJ, Miranda-Barrientos JA, Liu B, Zhang S, Wang HL, Vautier F, Ramakrishnan C, Kim YS, Fenno L, Deisseroth K, Morales M. 2020; Distinct signaling by ventral tegmental area glutamate, GABA, and combinatorial glutamate-GABA neurons in motivated behavior. Cell Rep. 32:108094. DOI: 10.1016/j.celrep.2020.108094. PMID: 32877676. PMCID: PMC7556367.


49. Bouarab C, Thompson B, Polter AM. 2019; VTA GABA neurons at the interface of stress and reward. Front Neural Circuits. 13:78. DOI: 10.3389/fncir.2019.00078. PMID: 31866835. PMCID: PMC6906177. PMID: 85dd5df8bfea41e08a65a841510005eb.


50. Sigel E, Steinmann ME. 2012; Structure, function, and modulation of GABAA receptors. J Biol Chem. 287:40224–40231. DOI: 10.1074/jbc.R112.386664. PMID: 23038269. PMCID: PMC3504738.
51. Benarroch EE. 2012; GABAB receptors: structure, functions, and clinical implications. Neurology. 78:578–584. DOI: 10.1212/WNL.0b013e318247cd03. PMID: 22351795.


52. van Zessen R, Phillips JL, Budygin EA, Stuber GD. 2012; Activation of VTA GABA neurons disrupts reward consumption. Neuron. 73:1184–1194. DOI: 10.1016/j.neuron.2012.02.016. PMID: 22445345. PMCID: PMC3314244.


53. Tan KR, Yvon C, Turiault M, Mirzabekov JJ, Doehner J, Labouèbe G, Deisseroth K, Tye KM, Lüscher C. 2012; GABA neurons of the VTA drive conditioned place aversion. Neuron. 73:1173–1183. DOI: 10.1016/j.neuron.2012.02.015. PMID: 22445344. PMCID: PMC6690362.


54. Aosaki T, Tsubokawa H, Ishida A, Watanabe K, Graybiel AM, Kimura M. 1994; Responses of tonically active neurons in the primate's striatum undergo systematic changes during behavioral sensorimotor conditioning. J Neurosci. 14:3969–3984. DOI: 10.1523/JNEUROSCI.14-06-03969.1994. PMID: 8207500. PMCID: PMC6576948.


55. Pan WX, Brown J, Dudman JT. 2013; Neural signals of extinction in the inhibitory microcircuit of the ventral midbrain. Nat Neurosci. 16:71–78. DOI: 10.1038/nn.3283. PMID: 23222913. PMCID: PMC3563090.


56. Eshel N, Bukwich M, Rao V, Hemmelder V, Tian J, Uchida N. 2015; Arithmetic and local circuitry underlying dopamine prediction errors. Nature. 525:243–246. Erratum in: Nature. 2015;527:398. DOI: 10.1038/nature14855. PMID: 26322583. PMCID: PMC4567485.


57. Wakabayashi KT, Feja M, Baindur AN, Bruno MJ, Bhimani RV, Park J, Hausknecht K, Shen RY, Haj-Dahmane S, Bass CE. 2019; Chemogenetic activation of ventral tegmental area GABA neurons, but not mesoaccumbal GABA terminals, disrupts responding to reward-predictive cues. Neuropsychopharmacology. 44:372–380. DOI: 10.1038/s41386-018-0097-6. PMID: 29875446. PMCID: PMC6300533.


58. Li Y, Li CY, Xi W, Jin S, Wu ZH, Jiang P, Dong P, He XB, Xu FQ, Duan S, Zhou YD, Li XM. 2019; Rostral and caudal ventral tegmental area GABAergic inputs to different dorsal raphe neurons participate in opioid dependence. Neuron. 101:748–761.e5. Erratum in: Neuron. 2021;109:3893-3894. DOI: 10.1016/j.neuron.2018.12.012. PMID: 30638902.


59. Tritsch NX, Oh WJ, Gu C, Sabatini BL. 2014; Midbrain dopamine neurons sustain inhibitory transmission using plasma membrane uptake of GABA, not synthesis. Elife. 3:e01936. DOI: 10.7554/eLife.01936. PMID: 24843012. PMCID: PMC4001323. PMID: 186232619a6b452a859d2b870a7b50b9.


60. Kim JI, Ganesan S, Luo SX, Wu YW, Park E, Huang EJ, Chen L, Ding JB. 2015; Aldehyde dehydrogenase 1a1 mediates a GABA synthesis pathway in midbrain dopaminergic neurons. Science. 350:102–106. DOI: 10.1126/science.aac4690. PMID: 26430123. PMCID: PMC4725325.


61. Tritsch NX, Ding JB, Sabatini BL. 2012; Dopaminergic neurons inhibit striatal output through non-canonical release of GABA. Nature. 490:262–266. DOI: 10.1038/nature11466. PMID: 23034651. PMCID: PMC3944587.


62. Zweifel LS, Fadok JP, Argilli E, Garelick MG, Jones GL, Dickerson TM, Allen JM, Mizumori SJ, Bonci A, Palmiter RD. 2011; Activation of dopamine neurons is critical for aversive conditioning and prevention of generalized anxiety. Nat Neurosci. 14:620–626. DOI: 10.1038/nn.2808. PMID: 21499253. PMCID: PMC3083461.


63. Bariselli S, Glangetas C, Tzanoulinou S, Bellone C. 2016; Ventral tegmental area subcircuits process rewarding and aversive experiences. J Neurochem. 139:1071–1080. DOI: 10.1111/jnc.13779. PMID: 27546491.


64. DeGroot SR, Zhao-Shea R, Chung L, Klenowski PM, Sun F, Molas S, Gardner PD, Li Y, Tapper AR. 2020; Midbrain dopamine controls anxiety-like behavior by engaging unique interpeduncular nucleus microcircuitry. Biol Psychiatry. 88:855–866. DOI: 10.1016/j.biopsych.2020.06.018. PMID: 32800629. PMCID: PMC8043246.


Fig. 1
Distinct cell populations in ventral tegmental area (VTA) process motivated behavior.
(A) Three cell populations in VTA; dopaminergic neurons (TH-positive), glutamatergic neurons (VGluT2-positive), and GABAergic neurons (VGAT-positive), with each afferent projection regarding the motivated behavior. (B) Distribution of each cell population in VTA. PFC, prefrontal cortex; NAc, nucleus accumbens; VP, ventral pallidum; MSNs, medium spiny neurons; LHb, lateral habenula; DRN, dorsal raphe nucleus.
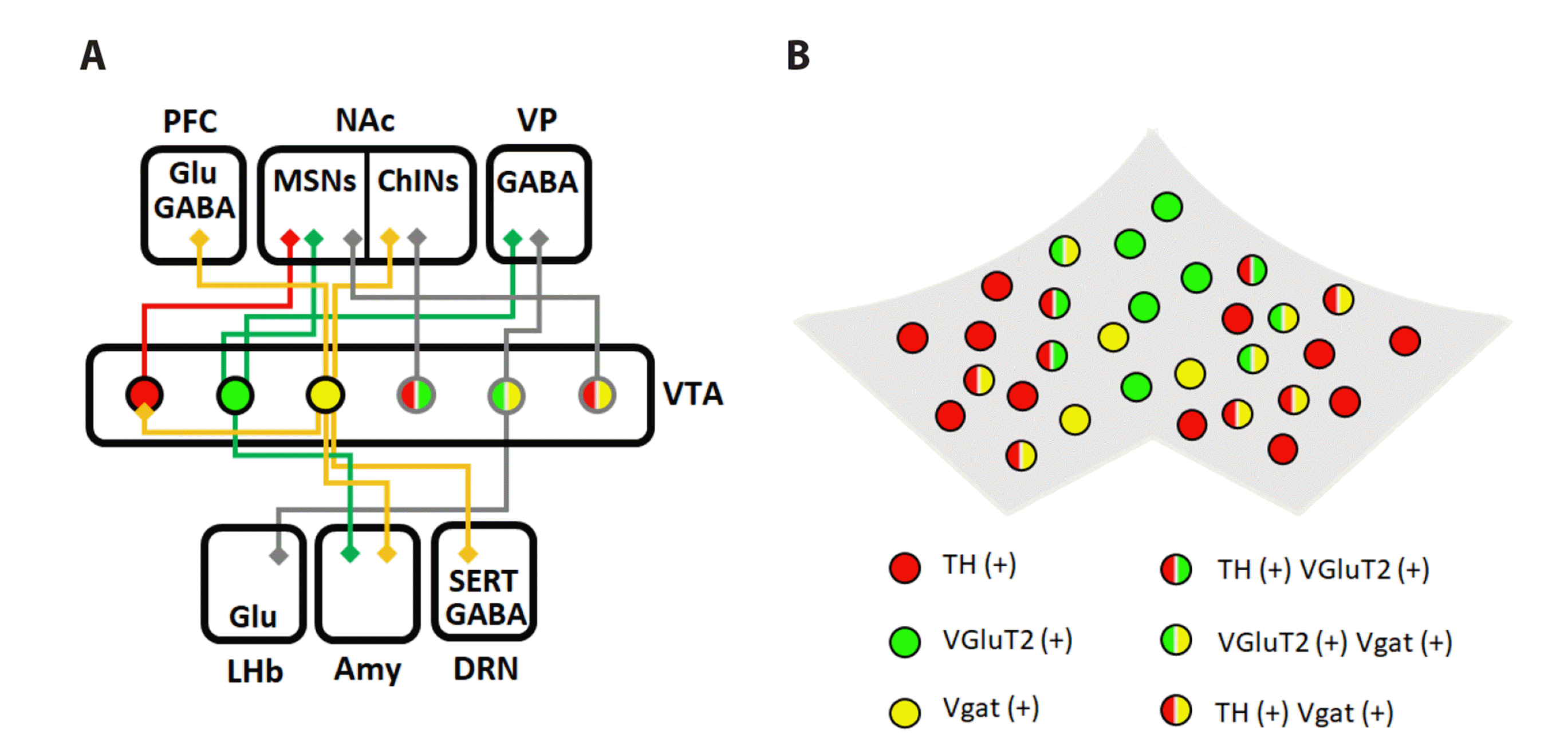