INTRODUCTION

METHODS
Design and specifications of the IBI
Experimental design of IBI implantation and stem cell administration in beagles
![]() | Fig. 1Scheme of IBI implantation and stem cell administration. In each group, MRI was taken at 1) baseline (after marker screw insertion), 2) pre-injection (before stem cell administration), and 3) post-injection (after stem cell administration).IBI = IntraBrain Injector, MRI = magnetic resonance imaging.
|
Magnetic resonance imaging (MRI) protocol
Determination of trajectory toward the target point
![]() | Fig. 2Determination of insertion trajectory and design of the surgical guide and adaptor. (A) The target area (the hippocampus in this study, marked in purple) was determined based on coronal view MRI after screw insertion. To determine the ideal trajectory, 3D-rendered images of the skull and brain were reconstructed. (B) An ideal insertion trajectory was considered one that allowed insertion of the IBI into the gyrus with minimal muscle detachment. (C) The surgical guide and adaptor were designed based on the location of three marker screw landmarks on the skull, reflecting the skull curvature. (D) The surgical guide (blue) was positioned to exactly locate the drilling point of the burr hole. (E) The adaptor was positioned in line with the burr hole, not only to fill the gap between the flat bottom surface of IBI and the curved skull surface, but to prevent the IBI from invading the brain surface.IBI = IntraBrain Injector, MRI = magnetic resonance imaging, MR = magnetic resonance.
|
Design of the adaptor and the surgical guide for precise injection targeting using 3D printing
Surgical procedure used for IBI implantation
![]() | Fig. 3Surgical procedures for IBI implantation in beagles. An illustration of operational procedures for the IBI. (A) Skin and muscles above the skull were incised minimally along the midline. Location of the screws on the skull that had been installed before IBI implantation was confirmed. Beagles were placed on a stereotaxic frame for the surgery. (B) A 3D-printed surgical guide (coloured in green) was placed on the skull, according to the location of marker screws. (C) Using the surgical guide, a burr hole (coloured in red) was drilled. (D) A round-shaped adapter (coloured in blue) was anchored above the burr hole. (E) The IBI was placed on the adapter.IBI = IntraBrain Injector.
|
Confirmation of IBI placement after implantation
Blood collection and analysis
Administration of ferumoxytol-labeled human umbilical cord blood-derived mesenchymal stem cells (hUCB-MSCs)
Ethics statement

RESULTS
Target accuracy of IBI implantation
![]() | Fig. 4Validation of target approach accuracy. Validation of target accuracy is shown in 3D rendered views and coronal MRI in (A) group 1 (six injections), (B) group 2 (three injections), and (C) group 3 (single injection). The white arrow shows a signal indicative of haemorrhage after surgery in the MRI of Subject 6-1.MRI = magnetic resonance imaging.
|
Surgery-related safety
![]() | Fig. 5MRI used for evaluation. MRI results of pre- and post-injection in (A) six-injection group, (B) three-injection group, and (C) single injection group.MRI = magnetic resonance imaging.
Failed results marked with superscript ‘a’ indicate an uncertain signal and those marked with superscript ‘b’ indicate signals detected further than 2 mm from the target area.
|
Table 1
Complete blood count results for the three groups of beagles
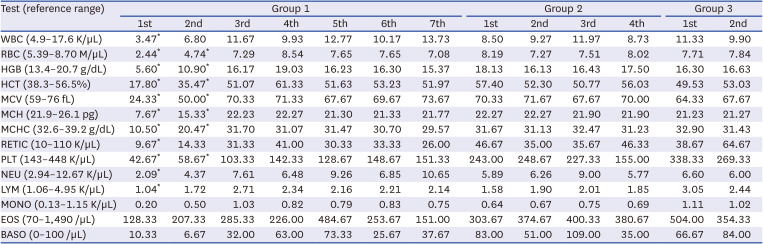
Imaging confirmation of repeated ferumoxytol-labeled MSC injections
Six-injection group

DISCUSSION
Table 2
Summary of the type and function of previously reported medical devices and the IBI
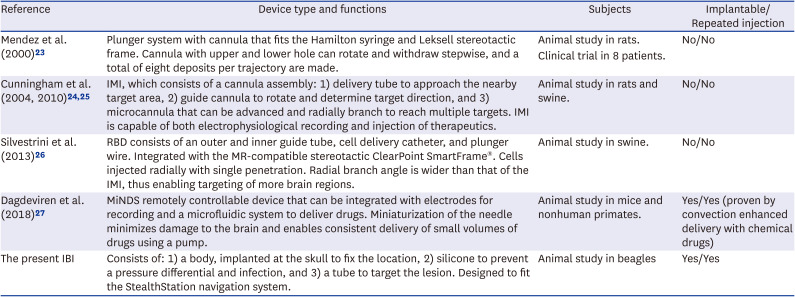
Reference | Device type and functions | Subjects | Implantable/Repeated injection |
---|---|---|---|
Mendez et al. (2000) 23 | Plunger system with cannula that fits the Hamilton syringe and Leksell stereotactic frame. Cannula with upper and lower hole can rotate and withdraw stepwise, and a total of eight deposits per trajectory are made. | Animal study in rats. | No/No |
Clinical trial in 8 patients. | |||
Cunningham et al. (2004, 2010) 24 , 25 | IMI, which consists of a cannula assembly: 1) delivery tube to approach the nearby target area, 2) guide cannula to rotate and determine target direction, and 3) microcannula that can be advanced and radially branch to reach multiple targets. IMI is capable of both electrophysiological recording and injection of therapeutics. | Animal study in rats and swine. | No/No |
Silvestrini et al. (2013) 26 | RBD consists of an outer and inner guide tube, cell delivery catheter, and plunger wire. Integrated with the MR-compatible stereotactic ClearPoint SmartFrame®. Cells injected radially with single penetration. Radial branch angle is wider than that of the IMI, thus enabling targeting of more brain regions. | Animal study in swine. | No/No |
Dagdeviren et al. (2018) 27 | MiNDS remotely controllable device that can be integrated with electrodes for recording and a microfluidic system to deliver drugs. Miniaturization of the needle minimizes damage to the brain and enables consistent delivery of small volumes of drugs using a pump. | Animal study in mice and nonhuman primates. | Yes/Yes (proven by convection enhanced delivery with chemical drugs) |
The present IBI | Consists of: 1) a body, implanted at the skull to fix the location, 2) silicone to prevent a pressure differential and infection, and 3) a tube to target the lesion. Designed to fit the StealthStation navigation system. | Animal study in beagles | Yes/Yes |
