Abstract
The liver is a vital organ that regulates systemic energy metabolism and many physiological functions. Nonalcoholic fatty liver disease (NAFLD) is the commonest cause of chronic liver disease and end-stage liver failure. NAFLD is primarily caused by metabolic disruption of lipid and glucose homeostasis. Serotonin (5-hydroxytryptamine [5-HT]) is a biogenic amine with several functions in both the central and peripheral systems. 5-HT functions as a neurotransmitter in the brain and a hormone in peripheral tissues to regulate systemic energy homeostasis. Several recent studies have proposed various roles of 5-HT in hepatic metabolism and inflammation using tissue-specific knockout mice and 5-HT-receptor agonists/antagonists. This review compiles the most recent research on the relationship between 5-HT and hepatic metabolism, and the role of 5-HT signaling as a potential therapeutic target in NAFLD.
The liver is an essential organ that regulates systemic energy metabolism and several physiological functions, including major macronutrient metabolism, immunomodulation, lipid metabolism and cholesterol homeostasis, and detoxification of several harmful reactive chemical species [1]. Thus, hepatic dysfunction is associated with systemic diseases such as cardiovascular disease, chronic kidney disease, obesity, and type 2 diabetes mellitus [2].
Nonalcoholic fatty liver disease (NAFLD), the commonest cause of chronic liver disease and end-stage liver failure [3], is defined as the accumulation of excess fat in the liver and includes a wide range conditions from simple fat accumulation in hepatic cells to nonalcoholic steatohepatitis, liver cirrhosis, and, finally, liver cancer [2]. Thus, NAFLD is a multisystem disease that is associated with both cardiovascular and hepatic morbidity and mortality [2,4]. The development and progression of NALFD are associated with multiple pathogenic mechanisms, such as fatty acid accumulation due to high lipid intake, insulin resistance-related upregulation of lipogenesis, lipid peroxidation-mediated liver injury, and elevated oxidative or endoplasmic reticulum stress [5,6], and metabolic disruption of lipid and glucose homeostasis is a major contributor of NAFLD pathogenesis [5]. Therefore, the restoration of hepatic metabolic homeostasis is a promising therapeutic approach for the treatment of NAFLD.
Serotonin (5-hydroxytryptamine [5-HT]) is a biogenic amine that plays numerous roles, through the regulation of systemic energy metabolism, in central and peripheral systems [7] as a neurotransmitter in the brain and as a hormone in peripheral tissues, respectively [8]. This review was conducted with the aim to compile the findings of recent studies of the relationship between 5-HT and hepatic metabolism and to discuss the role of 5-HT signaling as a potential treatment for NAFLD.
L-tryptophan is an essential amino acid, and more than 90% of tryptophan is metabolized in the kynurenine pathways, and others are used to make 5-HT, melatonin, tryptamine, and indole-pyruvic acid [9]. 5-HT synthesis, both central and peripheral, is initiated through tryptophan hydroxylase (TPH)-mediated tryptophan hydroxylation that is closely related to tryptophan availability, kynurenine synthesis, and TPH, which is the rate-limiting enzyme [8] and occurs in two isoforms: TPH1 is mainly expressed in peripheral tissues and, centrally, TPH1 is mainly expressed in the pineal gland and at extremely low levels in the rest of the central nervous system (CNS) [10]; TPH2 is the predominant enzyme isoform for neuronal 5-HT synthesis in the CNS and the enteric nervous system (ENS) [7]. As 5-HT does not cross the blood–brain barrier, changes in activities of TPH1 and TPH1 alters 5-HT levels in peripheral tissues and CNS, respectively.
From tryptophan, TPH generates 5-hydroxytryptophan (5-HTP), which is then converted to 5-HT by aromatic acid decarboxylase (AADC). The 5-HT transporter (serotonin transporter [SERT]) facilitates 5-HT reuptake, and monoamine oxidase (MAO)-A catalyzes the oxidative deamination of 5-HT. Both SERT and MAO-A activities are important factors to determine 5-HT levels in the target organs [11]. Furthermore, 5-HT can be converted to N-acetyl-serotonin by arylalkylamine N-acetyltransferase and, subsequently, to melatonin by hydroxyindole O-methyltransferase in the pineal gland and retina [12]. Moreover, indoleamine 2,3-dioxygenase (IDO) metabolizes tryptophan, thereby allowing it to enter the kynurenine pathway, which accounts for approximately 95% of dietary tryptophan degradation [9].
Through receptor signaling pathways, 5-HT modulates various physiological and pathological processes. Most of the 5-HT-related biological processes are mediated through more than fourteen 5-HT receptors that are categorized into seven families [7], of which six are G-protein-coupled receptors and one is a ligand-gated cation channel (serotonin receptor 3 [HTR3]) (Table 1, Fig. 1) [7].
Central 5-HT plays a major role in appetite control and systemic energy metabolism [13]. Several studies have elucidated the roles of 5-HT and its receptors in the CNS, and investigations in 5-HT receptor knockout (KO) mice have provided evidence that supports the role of central 5-HT in controlling appetite and/or bodyweight [14]. Via the 5-HT1B receptor, 5-HT stimulates the release of α-melanocyte-stimulating hormone in the proopiomelanocortin (POMC) neuron and suppresses the secretion of agouti-related protein (AgRP) in the orexigenic neuropeptide Y (NPY)/AgRP neuron [15], with a resultant decrease in appetite. The 5-HT2C receptor agonist lorcaserin (Belviq, Eisai Inc., Tokyo, Japan), which showed significant bodyweight reduction via appetite suppression, was approved in 2012 for the treatment of obesity by the Food and Drug Administration (FDA) [16] and saw widespread use as an anti-obesity medication until the FDA requested its withdrawal in 2020 due to an increased risk of cancer [17].
Central 5-HT signaling increases energy expenditure via the induction of thermogenic activity in brown adipose tissue (BAT) [14]. TPH2-positive neurons are a component of the neural circuitry between the brain and BAT [18]. The rostral raphé pallius, which regulates sympathetically mediated metabolism and the thermogenic activity of BAT, contains 5-HT neurons [19]. Microinjections of the 5-HT receptor antagonist, methysergide, into the intermediolateral cell column of the rat spinal cord suppressed cold-induced thermogenic activity in the BAT [19]. Central 5-HT deficiency resulted in the loss of thermoregulation and decreased the uncoupling protein 1 (UCP1) content in both BAT and inguinal white adipose tissue (WAT) [20]. Furthermore, through changes in the autonomic nervous system (ANS) and hormonal secretions, central 5-HT regulates peripheral glucose and lipid homeostasis [13], and POMC and AgRP neurons regulate glucose and lipid metabolism [13]. Thus, 5-HT2C receptor agonists regulate energy and glucose homeostasis and appetite via POMC neurons [21], and the AgRP neurons regulate hepatic glucose production [22].
The majority (>95%) of 5-HT is synthesized by TPH1 in enterochromaffin cells of gut and stored in platelets [7]. The major peripheral organs, such as the heart, adipose tissue, pancreatic islets, and skeletal muscle, contain TPH1 and can synthesize 5-HT [23–26]. Furthermore, the ENS can produce 5-HT from TPH2 [27]. Thus, 5-HT can regulate the metabolic function of peripheral organs through autocrine/paracrine pathways.
Through 5-HT receptor transduction in the ENS, 5-HT regulates gut motility through the modulation of muscular peristaltic activity via motor and sensory functions. Submucosal and myenteric neurons that are involved in intestinal peristalsis, secretion, and sensation are regulated by 5-HT3 and 5-HT4 [28]. Ondansetron, a 5-HT3 receptor antagonist that is widely used to prevent nausea and vomiting, can induce side effects such as constipation and ileus. The 5-HT4 receptor accelerates propulsive motility and reduces visceral pain in the large intestine [29]. In vitro, a 5-HT4 receptor agonist can increase enteric neuronal development and survival [30].
Recent studies have suggested a possible relationship between gut microbiota and 5-HT signaling in the gastrointestinal tract. Compared to WT mice, Tph1-KO mice had different gut microbiota composition, which conferred a protective effect that resulted in a lower susceptibility to colitis [31], and which suggested that 5-HT regulates gut microbiota composition. In contrast, gut microbiota alters 5-HT levels in the colon and blood by directly regulating gastrointestinal tryptophan metabolism [32].
Pancreatic islets are important for glycemic control because they comprise α- and β-cells that secrete glucagon and insulin, respectively. During pregnancy, pancreatic β-cells synthesize 5-HT, which increases β-cell mass and glucose-stimulated insulin secretion via 5-HT2B and 5-HT3 receptor signaling, respectively [26,33]. In addition, 5-HT regulates insulin secretion in a diet-induced insulin resistance state [34] and controls adult β-cell mass by stimulating perinatal β-cell proliferation [35]. Furthermore, 5-HT regulates energy metabolism in adipose tissue. During fasting conditions, both lipolysis in adipose tissue and gluconeogenesis in the liver are increased. Sumara et al. [36] discovered that fasting increased the level of gut-derived 5-HT (GDS), which promoted lipolysis in WAT via 5-HT2b receptor signaling.
Tph1 and Tph2 double-KO mice, as well as Tph1-KO mice, have lower body weight [37,38]. In contrast, the body weights of gut-specific Tph1-KO mice are comparable to those of WT control mice [36]. Intriguingly, on a high-fat diet (HFD), adipose tissue-specific Tph1-KO mice had less weight gain than WT mice [24]. The HFD increased 5-HT levels in WAT with upregulated Tph1 expression, and 5-HT subsequently upregulated lipid accumulation in WAT via 5-HT2A receptor-induced lipogenesis [24,39]. These findings imply that 5-HT, in addition to GDS, possibly plays a role in the regulation of energy homeostasis, especially in WAT.
Furthermore, recent studies suggest possible roles of 5-HT in BAT. On an HFD, Tph1-KO mice showed increased energy expenditure when a peripheral TPH inhibitor (LP-533401) was used to confirm the obesogenic actions of peripheral 5-HT [24,40]. In adipocyte-specific Tph1-KO mice, 5-HT depletion induced Ucp1 and Dio2 expression in the BAT and subcutaneous WAT [24]. In brown fat, the 5-HT3 receptor is important for diet-induced thermogenesis, which significantly increased in Htr3a-KO (whole-body KO) mice fed an HFD [31]. Therefore, this lean phenotype could be attributed to either central or peripheral 5-HT effects. Future research is required to identify the primary 5-HT receptor that regulates BAT thermogenesis.
The hepatocyte is unable to produce 5-HT; however, cholangiocytes and hepatic stellate cells (HSC) produce 5-HT in the liver [41]. Intrahepatic 5-HT-containing neurons and 5-HT receptors are distributed in the ANS, on branches of the hepatic artery, portal vein, bile duct, and the connective tissue of the interlobar septa [42]. Thus, neuronal signals from the ANS as well as circulating 5-HT from the gut regulates hepatic biology and metabolic functions, such as hepatic blood flow and regeneration.
In animal studies, 5-HT increased portal resistance in dogs [43] whereas the 5-HT2 receptor antagonist ketanserin reduced portal hypertension in rats [44]. The 5-HT2 receptor antagonist LY53857 reduced hepatic sinusoidal blood flow by acting on the HSC, which has both 5-HT2A and 5-HT2B receptors [45]. Furthermore, Kulinskii et al. [46,47] reported a major role of 5-HT in hepatic regeneration and wound healing using a murine partial hepatectomy (PHx) model. Tph1-KO mice failed to regenerate the liver after PHx, and 5-HT2 and 5-HT7 antagonists suppressed liver regeneration in post-PHx rats [48]. In a murine PHx model, the 5-HT2A receptor mediated liver regeneration [49]. The fibrogenic HSC have 5-HT2B receptor, and a 5-HT2B antagonist attenuated hepatic fibrosis and improved hepatic function in a murine chronic liver inflammation model [50]. Additionally, a recent study in a murine PHx model suggested a novel role of 5-HT, based on the activation the Hippo signaling pathway, in promoting hepatic regeneration [51].
Selective serotonin reuptake inhibitors (SSRIs) and serotonin noradrenaline reuptake inhibitors (SNRIs) reduced the intraplatelet 5-HT concentration. A recent clinical study reported that perioperative SSRI/SNRI intake was significantly associated with the high incidence of morbidity and postoperative hepatic dysfunction [52].
The liver plays an important role in the regulation of both glucose and lipid homeostasis. To maintain plasma glucose levels during fasting, the liver increases glycogenolysis and gluconeogenesis. Sumara et al. [36] discovered that, via 5-HT2B receptor signaling during fasting, GDS promotes gluconeogenesis and inhibits hepatic glucose uptake. Furthermore, GDS inhibits intrahepatocyte glucose uptake via a glucose transporter 2-dependent mechanism.
In contrast, several studies found contradictory results. 5-HT boosted hepatic glucose uptake and increased intrahepatic fat content in a dog model [53]. In ob/ob mice, the duodenal 5-HT content increased, and treatment with a 5-HT3 receptor antagonist reduced the elevated 5-HT levels while increasing SERT activity in the duodenum. Moreover, the 5-HT3 receptor antagonist reduced fat content, inflammation, and necrosis in these mice [54]. Crane et al. [40] discovered an intrahepatic role for peripheral 5-HT in mice fed an HFD: WT mice became obese and developed fatty liver disease when fed an HFD, whereas Tph1-KO mice did not develop fatty liver on an HFD and had reduced hepatic fat accumulation.
Recently, Choi et al. [55] proposed 5-HT as a therapeutic target for NAFLD. Gut-specific Tph1-KO and liver-specific Htr2a-KO mice were both resistant to HFD-induced hepatic steatosis. In addition, the 5-HT2A antagonist sarpogrelate ameliorated hepatic steatosis in HFD-fed mice. Thus, GDS regulates hepatic lipid accumulation through 5-HT2A receptor signaling. Gene set enrichment analysis (GSEA) of the liver transcriptomes of HFD-fed liver-specific Htr2a-KO mice and WT littermates revealed that gene sets related to inflammation, fibrosis, and steatohepatitis were significantly downregulated in the liver of the former.
HSC are important cellular components of hepatic fibrosis and wound healing. 5-HT1A, 5-HT1F, 5-HT2A, 5-HT2B, and 5-HT7 receptors are expressed in rat and human HSC [56]. 5-HT works in tandem with platelet-derived growth factor to promote HSC proliferation [56]. In the diseased rat liver, 5-HT2B receptor was strongly associated with fibrotic tissue. 5-HT2 receptor antagonist treatment of HSC reduced their proliferation and increased their rate of apoptosis [56]. In human HSC lines, 5-HT2A receptor antagonists inhibited viability and wound healing [57]. Moreover, 5-HT2B receptor signaling activated HSC by increasing TGF-1 signaling [50]. Inhibition of the 5-HT2B receptor suppressed fibrogenesis and improved liver function in a murine carbon tetrachloride (CCl4)-induced cirrhosis model [50].
Genetics and molecular research using human samples to identify underlying mechanisms and novel therapeutic targets for NAFLD have revealed potential roles for 5-HT signaling in NAFLD. The mRNA expressions of 5-HT2A and 5-HT2B receptor in human omental adipose tissue were significantly increased in obese subjects compared to lean subjects, and these gene expressions were positively correlated with serum aspartate aminotransferase and alanine aminotransferase levels [58]. A recent single-center clinical study found that levels of 5-hydroxyindoleacetic acid (5-HIAA; a metabolite of 5-HT) were significantly associated with hepatic events in NAFLD patients [59]. Compared to women with morbid obesity with normal liver histology, women with morbid obesity and NAFLD had decreased hepatic 5-HT2A and 5-HT2B receptor mRNA expressions [60]. Pantano et al. [61] recently used total RNA sequencing data to examine the molecular characteristics of histologically normal and NAFLD human livers, and suggested that the 5-HT2B receptor gene is one of the top 26 candidate genes related to the severity of liver fibrosis.
In clinical practice, many drugs, such as tricyclic antidepressant (TCAs), SSRIs, SNRIs, and 5-HT receptor agonists/antagonists, that regulate 5-HT signaling are widely used (Table 2). Clinical studies have reported significant associations between the abovementioned drug classes and liver disease. Patients who received TCAs (imipramine, tianeptine) [62,63] and SNRIs (venlafaxine, duloxetine) developed hepatic steatosis [64,65]. A clinical study showed that fluoxetine, an SSRI, increased serum triglyceride levels and hepatic lipid accumulation [66,67]. A clinical trial on the cardiometabolic benefits of lorcaserin, a 5-HT2C receptor agonist, showed that lorcaserin significantly improved fatty liver in obese adults, and the effect remained significant even after controlling for fat mass and change in body weight [68].
The human body has two separate serotonergic systems: the central 5-HT and the peripheral 5-HT systems. The majority of research has concentrated on the mechanism whereby central 5-HT regulates mood and behavior. Increasingly, central 5-HT signaling has been used therapeutically for appetite suppression to facilitate weight loss. Central 5-HT regulates glucose and lipid metabolism as well as systemic energy metabolism by regulating the thermogenic activities of brown and beige fat [20,69,70]. Several recent studies have identified the role of peripheral 5-HT from gut enterochromaffin cells and other peripheral organs in the regulation of various biological functions and energy metabolism. Thus, increasing energy expenditure and improving insulin resistance by regulating peripheral 5-HT signaling have been proposed as a novel target for anti-obesity treatment [7,71]. 5-HT plays a major role in regulating hepatic circulation and regeneration, and peripheral 5-HT promotes fat deposition and lipogenesis. Moreover, 5-HT induces hepatic fibrosis and promotes HSC activation (Fig. 2). Inhibition of peripheral 5-HT signaling impaired the development of NAFLD in animal models [55,72]. In addition, clinical studies of drugs that affect 5-HT signaling have provided evidence of the pathophysiological importance of 5-HT in liver disease. Further molecular and clinical studies on the relationship between 5-HT and liver metabolism are needed to better understand the complex biology of 5-HT in the liver and to provide effective 5-HT- related therapeutic applications.
ACKNOWLEDGMENTS
This research was supported by the Basic Science Research Program through the National Research Foundation of Korea (NRF), funded by the Ministry of Education (2020R1C1-C1004999) and KHIDI-AZ Diabetes Research Program.
REFERENCES
1. Abai B. StatPearls. Treasure Island: StatPearls Publishing;2021. Chapter, Physiology, liver. https://www.ncbi.nlm.nih.gov/books/NBK535438
.
2. Fotbolcu H, Zorlu E. Nonalcoholic fatty liver disease as a multi-systemic disease. World J Gastroenterol. 2016; 22:4079–90.


3. Ferguson D, Finck BN. Emerging therapeutic approaches for the treatment of NAFLD and type 2 diabetes mellitus. Nat Rev Endocrinol. 2021; 17:484–95.


5. Chao HW, Chao SW, Lin H, Ku HC, Cheng CF. Homeostasis of glucose and lipid in non-alcoholic fatty liver disease. Int J Mol Sci. 2019; 20:298.


6. Pei K, Gui T, Kan D, Feng H, Jin Y, Yang Y, et al. An overview of lipid metabolism and nonalcoholic fatty liver disease. Biomed Res Int. 2020; 2020:4020249.


7. Oh CM, Park S, Kim H. Serotonin as a new therapeutic target for diabetes mellitus and obesity. Diabetes Metab J. 2016; 40:89–98.


8. Yabut JM, Crane JD, Green AE, Keating DJ, Khan WI, Steinberg GR. Emerging roles for serotonin in regulating metabolism: new implications for an ancient molecule. Endocr Rev. 2019; 40:1092–107.


9. Badawy AA. Kynurenine pathway of tryptophan metabolism: regulatory and functional aspects. Int J Tryptophan Res. 2017; 10:1178646917691938.


10. Nakamura K, Hasegawa H. Developmental role of tryptophan hydroxylase in the nervous system. Mol Neurobiol. 2007; 35:45–54.


11. Prah A, Purg M, Stare J, Vianello R, Mavri J. How monoamine oxidase a decomposes serotonin: an empirical valence bond simulation of the reactive step. J Phys Chem B. 2020; 124:8259–65.


12. Gauer F, Craft CM. Circadian regulation of hydroxyindole-O-methyltransferase mRNA levels in rat pineal and retina. Brain Res. 1996; 737:99–109.


13. Pissios P, Maratos-Flier E. More than satiety: central serotonin signaling and glucose homeostasis. Cell Metab. 2007; 6:345–7.


14. van Galen KA, Ter Horst KW, Serlie MJ. Serotonin, food intake, and obesity. Obes Rev. 2021; 22:e13210.
15. Namkung J, Kim H, Park S. Peripheral serotonin: a new player in systemic energy homeostasis. Mol Cells. 2015; 38:1023–8.


16. Vandenbussche N, Goadsby PJ. Lorcaserin safety in overweight or obese patients. N Engl J Med. 2019; 380:99.


17. Sharretts J, Galescu O, Gomatam S, Andraca-Carrera E, Hampp C, Yanoff L. Cancer risk associated with lorcaserin: the FDA’s review of the CAMELLIA-TIMI 61 Trial. N Engl J Med. 2020; 383:1000–2.


18. Cano G, Passerin AM, Schiltz JC, Card JP, Morrison SF, Sved AF. Anatomical substrates for the central control of sympathetic outflow to interscapular adipose tissue during cold exposure. J Comp Neurol. 2003; 460:303–26.


19. Madden CJ, Morrison SF. Endogenous activation of spinal 5-hydroxytryptamine (5-HT) receptors contributes to the thermoregulatory activation of brown adipose tissue. Am J Physiol Regul Integr Comp Physiol. 2010; 298:R776–83.


20. McGlashon JM, Gorecki MC, Kozlowski AE, Thirnbeck CK, Markan KR, Leslie KL, et al. Central serotonergic neurons activate and recruit thermogenic brown and beige fat and regulate glucose and lipid homeostasis. Cell Metab. 2015; 21:692–705.


21. Berglund ED, Liu C, Sohn JW, Liu T, Kim MH, Lee CE, et al. Serotonin 2C receptors in pro-opiomelanocortin neurons regulate energy and glucose homeostasis. J Clin Invest. 2013; 123:5061–70.


22. Könner AC, Janoschek R, Plum L, Jordan SD, Rother E, Ma X, et al. Insulin action in AgRP-expressing neurons is required for suppression of hepatic glucose production. Cell Metab. 2007; 5:438–49.


23. Chandran S, Guo T, Tolliver T, Chen W, Murphy DL, McPherron AC. Effects of serotonin on skeletal muscle growth. BMC Proc. 2012; 6(Suppl 3):O3.


24. Oh CM, Namkung J, Go Y, Shong KE, Kim K, Kim H, et al. Regulation of systemic energy homeostasis by serotonin in adipose tissues. Nat Commun. 2015; 6:6794.


25. Park H, Oh CM, Park J, Park H, Cui S, Kim HS, et al. Deletion of the serotonin receptor type 3A in mice leads to sudden cardiac death during pregnancy. Circ J. 2015; 79:1807–15.


26. Kim H, Toyofuku Y, Lynn FC, Chak E, Uchida T, Mizukami H, et al. Serotonin regulates pancreatic beta cell mass during pregnancy. Nat Med. 2010; 16:804–8.


27. Li Z, Chalazonitis A, Huang YY, Mann JJ, Margolis KG, Yang QM, et al. Essential roles of enteric neuronal serotonin in gastrointestinal motility and the development/survival of enteric dopaminergic neurons. J Neurosci. 2011; 31:8998–9009.


28. Shajib MS, Khan WI. The role of serotonin and its receptors in activation of immune responses and inflammation. Acta Physiol (Oxf). 2015; 213:561–74.


29. Hoffman JM, Tyler K, MacEachern SJ, Balemba OB, Johnson AC, Brooks EM, et al. Activation of colonic mucosal 5-HT(4) receptors accelerates propulsive motility and inhibits visceral hypersensitivity. Gastroenterology. 2012; 142:844–54.


30. Liu MT, Kuan YH, Wang J, Hen R, Gershon MD. 5-HT4 receptor-mediated neuroprotection and neurogenesis in the enteric nervous system of adult mice. J Neurosci. 2009; 29:9683–99.


31. Kwon YH, Wang H, Denou E, Ghia JE, Rossi L, Fontes ME, et al. Modulation of gut microbiota composition by serotonin signaling influences intestinal immune response and susceptibility to colitis. Cell Mol Gastroenterol Hepatol. 2019; 7:709–28.


32. Yano JM, Yu K, Donaldson GP, Shastri GG, Ann P, Ma L, et al. Indigenous bacteria from the gut microbiota regulate host serotonin biosynthesis. Cell. 2015; 161:264–76.


33. Ohara-Imaizumi M, Kim H, Yoshida M, Fujiwara T, Aoyagi K, Toyofuku Y, et al. Serotonin regulates glucose-stimulated insulin secretion from pancreatic β cells during pregnancy. Proc Natl Acad Sci U S A. 2013; 110:19420–5.


34. Kim K, Oh CM, Ohara-Imaizumi M, Park S, Namkung J, Yadav VK, et al. Functional role of serotonin in insulin secretion in a diet-induced insulin-resistant state. Endocrinology. 2015; 156:444–52.


35. Moon JH, Kim YG, Kim K, Osonoi S, Wang S, Saunders DC, et al. Serotonin regulates adult β-cell mass by stimulating perinatal β-cell proliferation. Diabetes. 2020; 69:205–14.


36. Sumara G, Sumara O, Kim JK, Karsenty G. Gut-derived serotonin is a multifunctional determinant to fasting adaptation. Cell Metab. 2012; 16:588–600.


37. Savelieva KV, Zhao S, Pogorelov VM, Rajan I, Yang Q, Cullinan E, et al. Genetic disruption of both tryptophan hydroxylase genes dramatically reduces serotonin and affects behavior in models sensitive to antidepressants. PLoS One. 2008; 3:e3301.


38. Gutknecht L, Araragi N, Merker S, Waider J, Sommerlandt FM, Mlinar B, et al. Impacts of brain serotonin deficiency following Tph2 inactivation on development and raphe neuron serotonergic specification. PLoS One. 2012; 7:e43157.


39. Shong KE, Oh CM, Namkung J, Park S, Kim H. Serotonin regulates de novo lipogenesis in adipose tissues through serotonin receptor 2A. Endocrinol Metab (Seoul). 2020; 35:470–9.


40. Crane JD, Palanivel R, Mottillo EP, Bujak AL, Wang H, Ford RJ, et al. Inhibiting peripheral serotonin synthesis reduces obesity and metabolic dysfunction by promoting brown adipose tissue thermogenesis. Nat Med. 2015; 21:166–72.


41. Omenetti A, Yang L, Gainetdinov RR, Guy CD, Choi SS, Chen W, et al. Paracrine modulation of cholangiocyte serotonin synthesis orchestrates biliary remodeling in adults. Am J Physiol Gastrointest Liver Physiol. 2011; 300:G303–15.


42. Ruddell RG, Mann DA, Ramm GA. The function of serotonin within the liver. J Hepatol. 2008; 48:666–75.


43. Richardson PD, Withrington PG. A comparison of the effects of bradykinin, 5-hydroxytryptamine and histamine on the hepatic arterial and portal venous vascular beds of the dog: histamine H1 and H2-receptor populations. Br J Pharmacol. 1977; 60:123–33.


44. Cummings SA, Groszmann RJ, Kaumann AJ. Hypersensitivity of mesenteric veins to 5-hydroxytryptamine- and ketanserin-induced reduction of portal pressure in portal hypertensive rats. Br J Pharmacol. 1986; 89:501–13.


45. Cummings JL, Cilento EV, Reilly FD. Hepatic microvascular regulatory mechanisms. XII. Effects of 5-HT2-receptor blockade on serotonin-induced intralobular hypoperfusion. Int J Microcirc Clin Exp. 1993; 13:99–112.
46. Kulinskii AS, Saratikov AS, Vstavskaia IuA, Udovitsina TI. Effect of substances altering the metabolism of endogenous serotonin on mitotic activity in the regenerating liver of mice. Farmakol Toksikol. 1983; 46:92–5.
47. Kulinskii VI, Saratikov AS, Vstavskaia IuA, Udovitsina TI. Receptors mediating serotonin-stimulating liver regeneration in mice. Biull Eksp Biol Med. 1983; 95:89–91.
48. Papadimas GK, Tzirogiannis KN, Mykoniatis MG, Grypioti AD, Manta GA, Panoutsopoulos GI. The emerging role of serotonin in liver regeneration. Swiss Med Wkly. 2012; 142:w13548.


49. Lesurtel M, Graf R, Aleil B, Walther DJ, Tian Y, Jochum W, et al. Platelet-derived serotonin mediates liver regeneration. Science. 2006; 312:104–7.


50. Ebrahimkhani MR, Oakley F, Murphy LB, Mann J, Moles A, Perugorria MJ, et al. Stimulating healthy tissue regeneration by targeting the 5-HT2B receptor in chronic liver disease. Nat Med. 2011; 17:1668–73.


51. Fang Y, Liu C, Shu B, Zhai M, Deng C, He C, et al. Axis of serotonin: pERK-YAP in liver regeneration. Life Sci. 2018; 209:490–7.


52. Starlinger P, Pereyra D, Hackl H, Ortmayr G, Braunwarth E, Santol J, et al. Consequences of perioperative serotonin reuptake inhibitor treatment during hepatic surgery. Hepatology. 2021; 73:1956–66.


53. Moore MC, DiCostanzo CA, Dardevet D, Lautz M, Farmer B, Neal DW, et al. Portal infusion of a selective serotonin reuptake inhibitor enhances hepatic glucose disposal in conscious dogs. Am J Physiol Endocrinol Metab. 2004; 287:E1057–63.


54. Haub S, Ritze Y, Ladel I, Saum K, Hubert A, Spruss A, et al. Serotonin receptor type 3 antagonists improve obesity-associated fatty liver disease in mice. J Pharmacol Exp Ther. 2011; 339:790–8.


55. Choi W, Namkung J, Hwang I, Kim H, Lim A, Park HJ, et al. Serotonin signals through a gut-liver axis to regulate hepatic steatosis. Nat Commun. 2018; 9:4824.


56. Ruddell RG, Oakley F, Hussain Z, Yeung I, Bryan-Lluka LJ, Ramm GA, et al. A role for serotonin (5-HT) in hepatic stellate cell function and liver fibrosis. Am J Pathol. 2006; 169:861–76.


57. Kim DC, Jun DW, Kwon YI, Lee KN, Lee HL, Lee OY, et al. 5-HT2A receptor antagonists inhibit hepatic stellate cell activation and facilitate apoptosis. Liver Int. 2013; 33:535–43.


58. Choi WG, Choi W, Oh TJ, Cha HN, Hwang I, Lee YK, et al. Inhibiting serotonin signaling through HTR2B in visceral adipose tissue improves obesity related insulin resistance. J Clin Invest. 2021. Oct. 7. [Epub]. https://doi.org/10.1172/JCI145331
.


59. Wegermann K, Howe C, Henao R, Wang Y, Guy CD, Abdelmalek MF, et al. Serum bile acid, vitamin E, and serotonin metabolites are associated with future liver-related events in nonalcoholic fatty liver disease. Hepatol Commun. 2021; 5:608–17.


60. Binetti J, Bertran L, Riesco D, Aguilar C, Martinez S, Sabench F, et al. Deregulated serotonin pathway in women with morbid obesity and NAFLD. Life (Basel). 2020; 10:245.


61. Pantano L, Agyapong G, Shen Y, Zhuo Z, Fernandez-Albert F, Rust W, et al. Molecular characterization and cell type composition deconvolution of fibrosis in NAFLD. Sci Rep. 2021; 11:18045.


62. Horst DA, Grace ND, LeCompte PM. Prolonged cholestasis and progressive hepatic fibrosis following imipramine therapy. Gastroenterology. 1980; 79:550–4.


63. Le Bricquir Y, Larrey D, Blanc P, Pageaux GP, Michel H. Tianeptine: an instance of drug-induced hepatotoxicity predicted by prospective experimental studies. J Hepatol. 1994; 21:771–3.
64. Levine B, Jenkins AJ, Queen M, Jufer R, Smialek JE. Distribution of venlafaxine in three postmortem cases. J Anal Toxicol. 1996; 20:502–5.


65. Vuppalanchi R, Hayashi PH, Chalasani N, Fontana RJ, Bonkovsky H, Saxena R, et al. Duloxetine hepatotoxicity: a case-series from the drug-induced liver injury network. Aliment Pharmacol Ther. 2010; 32:1174–83.


66. Ayyash A, Holloway AC. Fluoxetine-induced hepatic lipid accumulation is linked to elevated serotonin production. Can J Physiol Pharmacol. 2021; 99:983–8.


67. Pan SJ, Tan YL, Yao SW, Xin Y, Yang X, Liu J, et al. Fluoxetine induces lipid metabolism abnormalities by acting on the liver in patients and mice with depression. Acta Pharmacol Sin. 2018; 39:1463–72.


68. Tuccinardi D, Farr OM, Upadhyay J, Oussaada SM, Mathew H, Paschou SA, et al. Lorcaserin treatment decreases body weight and reduces cardiometabolic risk factors in obese adults: a six-month, randomized, placebo-controlled, double-blind clinical trial. Diabetes Obes Metab. 2019; 21:1487–92.


69. Cerpa V, Gonzalez A, Richerson GB. Diphtheria toxin treatment of Pet-1-Cre floxed diphtheria toxin receptor mice disrupts thermoregulation without affecting respiratory chemoreception. Neuroscience. 2014; 279:65–76.


70. Wyler SC, Lord CC, Lee S, Elmquist JK, Liu C. Serotonergic control of metabolic homeostasis. Front Cell Neurosci. 2017; 11:277.


Fig. 1
5-Hydroxytryptamine (5-HT) receptors and signaling pathways. The 5-HT1 and 5-HT5 receptors are Gi/Go-protein coupled receptors that inhibit adenylate cyclase (AC) and thus suppress the cyclic adenosine monophosphate (cAMP) downstream pathways. 5-HT2 receptors are Gq/G11-protein coupled receptors that activate phospholipase C, resulting in the activation of the inositol triphosphate (IP3) and diacylglycerol (DAG) downstream pathways. The only ligand-gated ion channel that can regulate membrane potential is the 5-HT3 receptor. The Gs-protein coupled receptors 5-HT4, 5-HT6, and 5-HT7 activate AC.
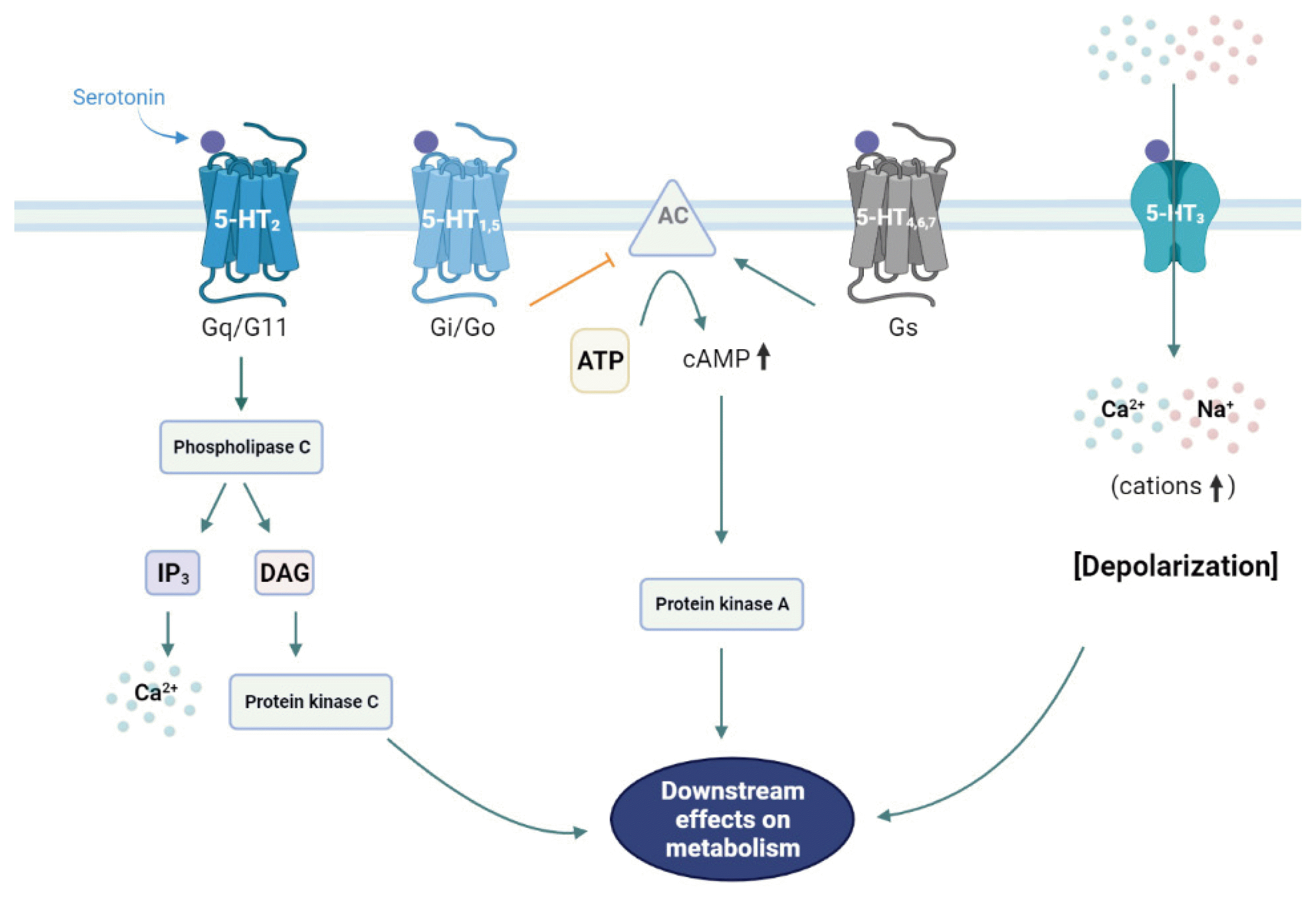
Fig. 2
Metabolic functions of 5-hydroxytryptamine (5-HT) in liver. Most peripheral 5-HTs are derived from enterochromaffin cells of the gut. 5-HT regulates hepatic fibrosis in hepatic stellate cells (HSCs) by activating HSC-produced transforming growth factor (TGF)-signaling. Through the 5-HT2B receptor, 5-HT promotes gluconeogenesis in hepatocytes by increasing the activity of fructose 1,6-bisphosphatase (FBPase) and glucose 6-phosphatase (G6pase). 5-HT2B receptor signaling also inhibits glucose uptake by promoting the breakdown of glucose transporter 2 (GLUT2). The activation of the sterol-regulatory-element-binding protein 1 (SREBP1) signaling pathway by the 5-HT2A receptor increases lipogenesis in the liver. ERK1/2, extracellular signal–regulated protein kinase.
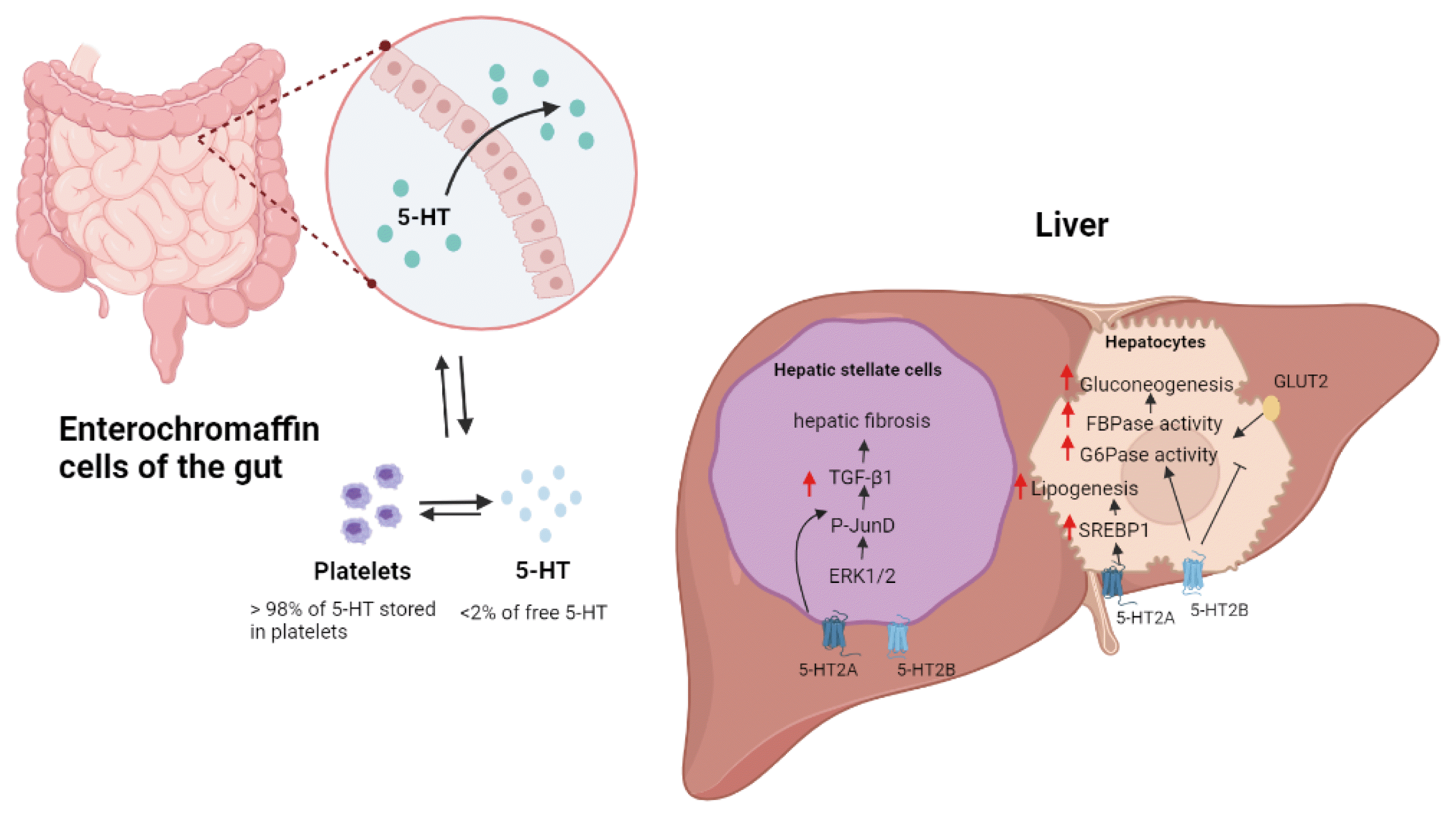
Table 1
5-HT Receptors, Structure, Transduction System, and Tissue Expression
Table 2
5-HT Receptor Agonists and Antagonists