Abstract
Background
Farnesoid X receptor (FXR), a bile acid–activated nuclear receptor, is a potent regulator of glucose and lipid metabolism as well as of bile acid metabolism. Previous studies have demonstrated that FXR deficiency is associated with metabolic derangements, including atherosclerosis and nonalcoholic fatty liver disease (NAFLD), but its mechanism remains unclear. In this study, we investigated the role of FXR in atherosclerosis and NAFLD and the effect of peroxisome proliferator-activated receptor (PPAR) agonists in mouse models with FXR deficiency.
Methods
En face lipid accumulation analysis, liver histology, serum levels of glucose and lipids, and mRNA expression of genes related to lipid metabolism were compared between apolipoprotein E (ApoE)−/− and ApoE−/−FXR−/− mice. The effects of PPARα and PPARγ agonists were also compared in both groups of mice.
Results
Compared with ApoE−/− mice, ApoE−/−FXR−/− mice showed more severe atherosclerosis, hepatic steatosis, and higher levels of serum cholesterol, low-density lipoprotein cholesterol, and triglycerides, accompanied by increased mRNA expression of FAS, ApoC2, TNFα, IL-6 (liver), ATGL, TGH, HSL, and MGL (adipocytes), and decreased mRNA expressions of CPT2 (liver) and Tfam (skeletal muscle). Treatment with a PPARα agonist, but not with a PPARγ agonist, partly reversed atherosclerosis and hepatic steatosis, and decreased plasma triglyceride levels in the ApoE−/−FXR−/− mice, in association with increased mRNA expression of CD36 and FATP and decreased expression of ApoC2 and ApoC3 (liver).
The development of atherosclerosis involves dyslipidemia, characterized by elevated low-density lipoprotein cholesterol (LDL-C), triglycerides [1], reduced high-density lipoprotein cholesterol (HDL-C), inflammation, and endothelial dysfunction [2]. These metabolic changes are often accompanied by increased fat deposition in the liver and consequent hepatitis, which is characterized by hepatocyte necrosis and fibrosis.
Farnesoid X receptor (FXR) is highly expressed in the liver, intestine, kidneys, and adrenal glands, and is known to be activated by bile acids, including cholic acid and chenodeoxycholic acid [3,4]. Activation of FXR decreases serum triglyceride levels, while FXR deficiency increases plasma levels of triglycerides and total cholesterol levels—both HDL-C, due to reduced clearance, and non-HDL-C—in animal models [5,6].
Based on studies on the effects of FXR on lipid metabolism, further investigations explored the pro-atherogenic effects of FXR. Whereas apolipoprotein E (ApoE)−/− mice are well established as a model of atherosclerosis, resulting from poor lipoprotein clearance and subsequent deposition of lipids in blood vessels [7], FXR−/− mice have not been able to demonstrate an atherosclerotic phenotype despite a proatherogenic profile of serum lipoproteins [8]. In a previous study comparing wild-type mice, ApoE−/− mice, FXR−/− mice, and ApoE−/−FXR−/− mice fed high fat/high cholesterol diets, plasma cholesterol levels showed sequentially increased levels in FXR−/−, ApoE−/−, and ApoE−/− FXR−/− mice, while plasma triglyceride levels were only significantly elevated in FXR−/− and ApoE−/−FXR−/− mice. Atherosclerotic plaques were detectable in ApoE−/− mice and were present at an augmented level in ApoE−/−FXR−/− mice, but not in FXR−/− mice, indicating that the double knockout genotype is necessary to study the role of FXR in atherosclerosis [9].
The activation of FXR by a synthetic agonist reversed diet-induced hypertriglyceridemia and elevations of non-HDL-C, while producing near-complete inhibition of aortic lesion formation in (low density lipoprotein receptor knock out (LDLR−/−) and ApoE−/− mice [10]. Another study also revealed that dual activation of the bile acid nuclear receptor FXR and G protein-coupled bile acid receptor 1 (GPBAR1 or TGR5) protected mice against atherosclerosis and reduced both circulating lipid levels and inflammation, strengthening the evidence for a relationship between FXR and atherosclerosis [11]. In addition, FXR downregulation has been implicated in the development of nonalcoholic fatty liver disease (NAFLD) [12], and FXR agonists were shown to improve hepatic fibrosis and inflammation in in vivo studies [13].
Peroxisome proliferator-activated receptors (PPARs) are well known modifiers of insulin sensitivity, lipid metabolism, and inflammation. PPARα is known to exert effects on multiple levels of fatty acid metabolism, and PPARγ is known to regulate adipose genes to promote lipolysis and clear triglyceride-rich lipoproteins, suggesting that they play favorable roles in various settings of altered lipid metabolism [14].
Thus, the aim of this study was to investigate the mechanisms of FXR-dependent atherosclerosis and NAFLD in detail, as well as the roles of PPARα and PPARγ agonists in this setting.
Both ApoE−/− and ApoE−/−FXR−/− mice (C57BL/6J) were provided by Professor Young Joo Park (Department of Internal Medicine, Seoul National University College of Medicine, Seoul, Korea). To generate ApoE−/−FXR−/− mice, homozygous knockout mice for ApoE or FXR were first generated by crossing heterozygous knock out mice for each gene. After confirming the selection by genotyping, the generated ApoE−/− mice and FXR−/− mice were subsequently crossed to generate ApoE−/−FXR−/− mice. The mice were fed with a Western diet (WD; 17% protein, 40% fat, 43% carbohydrate; Research Diets Inc., New Brunswick, NJ, USA). Pioglitazone and fenofibrate were administered via oral gavage at doses of 20 and 100 mg/kg for pioglitazone [15-18] and fenofibrate [19-21], respectively, to provide a moderately high dose based on safely tolerated doses in other mouse models in the previous literature for each drug.
At 8 weeks of age, the ApoE−/− mice (n=7) were kept on the WD for 12 weeks. The ApoE−/−FXR−/− mice (n=25) were divided into three groups and were kept on the WD alone (n=9), treated with pioglitazone (n=8), or treated with fenofibrate (n=8) for 12 weeks. Mice were weighed once every two weeks, and food consumption was monitored throughout the study. All animals were maintained at 23°C±2°C and 60%±10% humidity on a 12-hour light/dark cycle, and food and water were provided ad libitum. At the end of the study, mice were fasted for 8 hours, anesthetized, and sacrificed.
All procedures were conducted in accordance with the Guide for Standard Operation Procedures and with approval of the Institutional Animal Care and Use Committee (IACUC) in the Clinical Research Institute of Seoul National University Bundang Hospital (BA1407-156/030-03).
En face lipid accumulation was determined by removing aortas from the mice from the ileal bifurcation to the origin at the heart. The whole aorta was fixed in 4% phosphate buffered saline for 48 hours, cut longitudinally, pinned on a plate, and stained with Oil Red O to identify atherosclerotic lesions. Images were taken using a light microscope (Carl Zeiss, Oberkochen, Germany) and analyzed. Atherosclerotic lesions of the aorta were presented as the percentage of the sum of the Oil Red Opositive area to the total aortic surface area.
Liver tissue was fixed in 4% formaldehyde, dehydrated, embedded in paraffin, and sectioned (4 μm). Sections were stained with hematoxylin and eosin and Masson trichrome. The NAFLD activity score (NAS) and fibrosis score were calculated by an experienced pathologist. The NAS is defined as the sum of the scores for steatosis (0 to 3), lobular inflammation (0 to 3), and ballooning (0 to 2). The NAS ranges from 0 to 8 and a score equal to or greater than 5 is correlated with a diagnosis of nonalcoholic steatohepatitis. Fibrosis, which is thought to be a result of the disease rather than a reversible factor that indicates activity, was separately staged from 0 to 4 (0, none; 1, perisinusoidal or periportal; 1A, mild, zone 3, perisinusoidal; 1B, moderate, zone 3; perisinusoidal, 1C, portal/periportal; 3, bridging fibrosis; and 4, cirrhosis) [22].
Blood was obtained from the inferior vena cava after overnight fasting. Serum was prepared and stored at –80°C. Blood glucose levels were determined by a glucometer (ACCU-CHEK Active, Roche, Mannheim, Germany). Total cholesterol, triglyceride, HDL-C, and LDL-C levels were determined by the Beckman Coulter AU480 automatic biochemistry analysis system (Tokyo, Japan).
Total RNA was isolated from the liver, white adipose tissue, and gastrocnemius muscle using TRizol reagent (Ambion, Foster City, CA, USA), and cDNA was synthesized using Moloney murine leukemia virus (M-MLV) reverse transcriptase (Invitrogen, Carlsbad, CA, USA). Reverse-transcriptase quantitative polymerase chain reaction (RT-qPCR) was performed using the ABI 7500 Real Time PCR System (Applied Biosystems, Foster City, CA, USA) and amplification was achieved using the SYBR Premix Ex Taq polymerase (Takara, Otsu, Japan). Genespecific primers (Cosmo Genetech, Seoul, Korea) for selected genes were designed using Primer Express software (Applied Biosystems). The selected genes and their primer sequences are included in the supplementary material (Supplemental Table S1). All resulting data were analyzed using ABI 7500 software version 2.0.5 (Applied Biosystems).
The percentage of atherosclerosis was significantly higher in ApoE−/−FXR−/− mice than in ApoE−/− mice (5.9%±1.5% vs. 9.2%±2.4%, P=0.006) (Fig. 1A, B) even after weight adjustment (P=0.033). The increased atherosclerosis in ApoE−/− FXR−/− mice was reversed by PPARα agonist (fenofibrate) treatment (9.2%±2.4% vs. 4.4%±2.7% in WD controls, P=0.001) but not by PPARγ agonist (pioglitazone) treatment (9.2%±2.4% vs. 7.3%±1.5% in WD controls, P=0.216) (Fig. 1C).
ApoE−/−FXR−/− mice had higher serum levels of total cholesterol (1,091±176 mg/dL vs. 691±152 mg/dL, P=0.017), triglycerides (289±52 mg/dL vs. 179±49 mg/dL, P=0.016), and LDL-C (798±135 mg/dL vs. 574±79 mg/dL, P=0.008) than ApoE−/− mice. However, serum HDL-C levels did not differ significantly between the two groups (54±22 mg/dL vs. 47±27 mg/dL, P=0.683).
Treatment with fenofibrate decreased serum triglyceride level in ApoE−/−FXR−/− mice (190±73 mg/dL vs. 289 ±52 mg/dL in WD controls, P=0.028), whereas treatment with pioglitazone did not (310±35 mg/dL vs. 289 ±52 mg/dL in WD controls, P=0.874 in the post hoc analysis). Serum levels of total cholesterol, LDL-C, and HDL-C were unaffected by either treatment. Serum glucose levels were not different regardless of genetic background and treatment (Supplemental Table S2).
ApoE−/− mice showed predominantly microvesicular steatosis characterized by distended hepatocytes with foamy-appearing cytoplasm due to small lipid vesicles. In ApoE−/−FXR−/− mice, the liver showed predominantly macrovesicular steatosis characterized by distortion of the nucleus due to the large size of the lipid vesicles. ApoE−/−FXR−/− mice also demonstrated mild to moderate perivenular/perisinusoidal or periportal fibrosis (stages 1A to 2), in contrast to the ApoE−/− mice with no fibrosis (Fig. 2A, B, Supplemental Fig. S1A)
Treatment with fenofibrate significantly improved the degree of steatosis, but not the necroinflammatory changes or the NAS, which is a sum of scores assessing steatosis (score 0 to 3), lobular inflammation (0 to 3), and hepatocyte ballooning (0 to 2); thus ranging from 0 to 8. Treatment with pioglitazone improved neither steatosis nor the lobular necroinflammation (Fig. 2A, C, Supplemental Fig. S1B). No significant differences were observed in the degree of fibrosis among the ApoE−/−FXR−/− mice according to the treatment (data not shown).
Among the genes involved in fatty acid metabolism, the expression of fatty acid synthase (FAS) was significantly elevated and that of carnitine palmitoyltranferase 2 (CPT2) was significantly reduced in ApoE−/−FXR−/− mice compared to ApoE−/− mice (Fig. 3A, B). In the analysis of genes involved in triglyceride metabolism, ApoC2, which is known to activate lipoprotein lipase (LPL), was significantly decreased in ApoE−/−FXR−/− mice (Fig. 3C). An analysis of hepatic genes involving very LDL metabolism and LDL metabolism did not reveal any differences between ApoE−/− and ApoE−/−FXR−/− mice (Fig. 3D). The expression of genes for the inflammatory cytokines tumor necrosis factor-α (TNFα) and interleukin-6 (IL-6) was also significantly elevated in ApoE−/−FXR−/− mice (Fig. 3E). However, genes related to hepatic fibrosis were not altered (Fig. 3E).
The RT-qPCR analysis of genes involved in lipolysis in adipocytes showed markedly increased expression of adipocyte triglyceride lipase (ATGL), triglycerol hydrolase (TGH), hormone sensitive lipase (HSL), and monoglyceride lipase (MGL) (Fig. 3F). In the analysis of skeletal muscle genes, transcription factor A, mitochondrial (Tfam), which is involved in mitochondrial activation, was significantly decreased (Fig. 3G).
Fenofibrate treatment significantly increased the expression of CD36 (fatty acid translocase [FAT]) and fatty acid transport protein 1 (FATP1) in ApoE−/−FXR−/− mice (Fig. 4B), while not altering the genes related to fatty acid synthesis (Fig. 4A). Moreover, an analysis of genes involved in triglyceride hydrolysis showed that fenofibrate decreased levels of both ApoC2 and ApoC3, which are respectively activator and inhibitor genes of LPL, in ApoE−/−FXR−/− mice (Fig. 4C). Fenofibrate treatment did not affect the expression of genes involved in cholesterol metabolism, inflammation, hepatic fibrosis, adipocyte lipolysis, and mitochondrial activation (Fig. 4D-G). Pioglitazone treatment was not associated with improvement of any of the genes related to lipid metabolism (Fig. 4D-G).
This study showed that loss of FXR was associated with aggravation of atherosclerosis and hepatic steatosis in ApoE-deficient mice, which could be reversed by a PPARα agonist through induction of triglyceride hydrolysis and β-oxidation of fatty acids.
Treatment of ApoE−/−FXR−/− mice with a PPARα agonist (fenofibrate) showed a significant decrease in atherosclerotic lesions and hepatic fat accumulation, but treatment with a PPARγ agonist (pioglitazone) did not. Neither of the PPAR agonists caused significant changes in the degree of hepatic necroinflammation, ballooning, or fibrosis. Biochemically, reduction of serum triglyceride levels was the only factor that could account for the improvement in atherosclerosis and hepatic steatosis caused by the loss of FXR in fenofibrate-treated mice.
To explain the possible mechanisms for the above metabolic changes, we performed an RT-qPCR analysis of related genes. The examination of genes related to fatty acid synthesis, fatty acid uptake/catabolism, and triglyceride hydrolysis showed higher expression of FAS and lower expression of CPT2 and ApoC2 in ApoE−/−FXR−/− mice compared with ApoE−/− mice.
FAS is a known target gene of FXR, which is downregulated upon activation of FXR in the process of lipolysis [23,24]. The elevated expression of FAS in ApoE−/−FXR−/− mice implies that increased lipogenesis contributes to the elevated levels of triglycerides, in turn promoting atherosclerosis. CPTs are responsible for the mitochondrial uptake of the esterified fatty acids in preparation for β-oxidation. The decrease in CPT2 expression in ApoE−/−FXR−/− mice implies that decreased mitochondrial uptake of fatty acids might contribute to decreased fatty acid oxidation, resulting in a surplus of fatty acids in the liver and circulation. In addition, a significant reduction in the expression of ApoC2, which is an LPL activator gene, suggests the contribution of the loss of FXR to reduced triglyceride clearance.
The examination of genes related to inflammation showed higher expression of TNFα and IL-6 in ApoE−/−FXR−/− mice than in ApoE−/− mice, confirming the suppressive role of FXR on inflammation. The investigations of adipocyte lipolysis showed marked elevations of ATGL, TGH, HSL, and MGL in ApoE−/− FXR−/− mice. Previously, FXR agonists were shown to enhance insulin signaling in differentiated 3T3-L1 adipocytes, whereas FXR−/− mice showed decreased insulin sensitivity [6]. Insulin resistance in adipocytes promotes lipolysis and free fatty acid release in circulation [25]. These free fatty acids act on the liver to disrupt the actions of insulin on glucose while retaining its lipogenic actions, increasing de novo lipogenesis and re-esterification of free fatty acids in the form of triglycerides, which contributes to liver fat accumulation that further impairs insulin sensitivity and mitochondrial function. Accordingly, the analysis of mitochondrial activation genes measured in skeletal muscle tissue showed a significant decrease in Tfam in ApoE−/− FXR−/− mice, implying interdependent actions of insulin resistance, lipolysis, liver steatosis, and mitochondrial dysfunction resulting from the loss of FXR in ApoE−/− mice.
The above genes were again analyzed and compared after treatment with a PPAR agonist, and several changes were noted after PPARα agonist treatment. In the analysis of genes related to fatty acid synthesis and uptake/catabolism, increased expression of CD36 and FATP1, which are regulator genes for the cellular uptake of circulating fatty acids, was noted. In relation to TG clearance, ApoC2 and ApoC3, which are genes for positive and negative regulators of LPL, respectively, both showed lower levels of expression. The downregulation of ApoC2 and ApoC3, with a net effect of LPL activation, has been documented in previous studies. ApoC3 is a major constituent of the triglyceride-rich remnant lipoprotein, which impedes lipolysis by inhibiting LPL action. It can be presumed that the increased expression of ApoC3 with a reduction of the ApoC3/ApoC2 ratio may produce a net stimulatory effect on triglyceride hydrolysis [26-29]. The expression of genes related to adipocyte lipolysis, mitochondrial activation, and inflammation was not affected by fenofibrate treatment; this finding is compatible with the liver histology, which showed no improvements in lobular necroinflammation and NAS.
In short, decreased fatty acid oxidation and increased free fatty acid flux into the liver from adipocyte lipolysis, in addition to increased inflammation in association with insulin resistance, account for the metabolic changes caused by FXR loss. Improvements of these metabolic changes by fenofibrate treatment can be explained by the increased cellular uptake of fatty acids for catabolism in liver and LPL-mediated lipolysis by modulation of its regulators.
Previous experimental studies on atherosclerosis showed that FXR activation by agonists induced vascular smooth muscle cell apoptosis [30] and upregulated the expression of endothelial nitric oxide synthase in vitro [31]. In vivo studies using FXR agonists proved to reduce inflammatory cytokines in aortas of ApoE−/−FXR−/− mice and to reduce atherosclerotic lesions in LDLR−/− and ApoE−/− mice [10,32,33]. Dual activation of FXR with G-protein coupled receptor TGR5 also attenuated atherosclerosis both in LDLR−/− and ApoE−/− mice [11]. In addition, studies on NAFLD showed that FXR activation by natural and synthetic agonists improved hepatic steatohepatitis by reducing fatty acid synthesis via increasing the expressions of SHP, reducing the expression of sterol regulatory element-binding protein 1c (SREBP1c) [23], and increasing cholesterol efflux [34]. FXR agonist also produced a significant improvement of hepatic inflammation in mice [35] and liver fibrosis in humans [13].
Despite these promising results on the effect of FXR agonism in reducing cardiovascular morbidity and mortality, several factors preclude the development of FXR agonists as therapeutic agents. A clinical trial of obeticholic acid in NAFLD showed more episodes of pruritis, reversible heart failure, osteopenia, lower HDL-C levels, higher LDL-C levels, and more severe fatigue compared with placebo group despite improvement of liver histology through FXR agonism [36]. FXR agonists have also been found to cause HDL-C reduction in multiple in vitro studies, constituting a major obstacle to their development as metabolic enhancers [37-39].
In contrast, fenofibrate, a well-known PPARα agonist, has long been used in clinical settings for the treatment of patients with metabolic derangements focusing on triglyceride metabolism, and it has a predictable safety profile. Additionally, incubation of human hepatoma HepG2 cells with natural and synthetic FXR agonists both resulted in a significant induction of PPARα mRNA levels, suggesting crosstalk between FXR and PPARα [40]. These findings imply that fenofibrate may function as a potent alternative to FXR agonists as a therapeutic agent in patients with metabolic derangements related to FXR dysfunction, with lower risks of developing undesirable effects.
In this study, the improvement of serum triglyceride levels by fenofibrate, apart from persistent LDL-C levels, was able to reverse atherosclerosis and hepatic steatosis, although not to the degree of improving NAS. These changes were accompanied by increased fatty acid catabolism and LPL activation, reversing the changes brought by increased lipogenesis and decreased LPL activation in FXR deficiency. Although the results did not show direct crosstalk between FXR and PPARα, our study demonstrated that PPARα agonists can restore some of the metabolic derangements caused by FXR by working on common metabolic pathways to reduce plasma triglyceride levels, thereby improving atherosclerosis and NAFLD.
This study has limitations. First, our investigation focused mainly on triglyceride and free fatty acid metabolism, linking adipocyte lipolysis to the increased lipid accumulation in liver. A more thorough evaluation of fatty acid metabolism, including studies of free fatty acid trafficking with measurements of hepatic uptake of circulating free fatty acids, may better illustrate the links between liver and adipose tissues. Second, the development of atherosclerosis results from altered lipid metabolism in combination with insulin resistance, increased inflammatory response, and endothelial dysfunction. However, changes in inflammation and endothelial dysfunction in blood vessels were not examined. Lastly, the changes in fibroblast growth factor 15, which is known to play important metabolic roles in response to FXR activation [41], could not be measured in this study due to technical difficulties.
In conclusion, this study demonstrated that the additional loss of FXR increases atherosclerosis and NAFLD characterized by macrovesicular hepatic steatosis in ApoE-deficient mice. These changes are associated with combined dyslipidemia due to increased fatty acid synthesis in liver, and increased adipocyte lipolysis. Treatment with a PPARα agonist reversed the deleterious effects of ectopic fat accumulation by decreasing plasma triglyceride levels associated with enhanced triglyceride hydrolysis and β-oxidation of fatty acids.
Supplementary Information
Supplemental Table S1.
Primer Sequences for Reverse-Transcriptase Quantitative Polymerase Chain Reaction
Supplemental Table S2.
Weight Change, Food Intake, and Serum Glucose and Lipid Profiles
Supplemental Fig. S1.
(A) Comparison of nonalcoholic fatty liver disease (NAFLD) components between apolipoprotein E (ApoE)−/− and ApoE−/− farnesoid X receptor (FXR)−/− mice. (B) Comparison of NAFLD components in ApoE−/−FXR−/− mice fed with Western diet (WD) with or without pioglitazone or fenofibrate treatment. Error bars stand for standard deviation. aA significant difference between ApoE−/− and ApoE−/−FXR−/− mice; bA significant difference between ApoE−/−FXR−/− mice with no treatment and ApoE−/−FXR−/− mice treated with fenofibrate
ACKNOWLEDGMENTS
This article is based on author Yenna Lee’s Ph.D. thesis: Yenna Lee. (2018). The effect of PPAR agonists on atherosclerosis and non-alcoholic fatty liver disease in ApoE−/−FXR−/− mice (doctoral dissertation, Seoul National University, Seoul, Korea). Retrieved from https://s-space.snu.ac.kr/handle/10371/143308.
REFERENCES
1. Gautier T, de Haan W, Grober J, Ye D, Bahr MJ, Claudel T, et al. Farnesoid X receptor activation increases cholesteryl ester transfer protein expression in humans and transgenic mice. J Lipid Res. 2013; 54:2195–205.


2. Hansson GK. Inflammation, atherosclerosis, and coronary artery disease. N Engl J Med. 2005; 352:1685–95.


3. Makishima M, Okamoto AY, Repa JJ, Tu H, Learned RM, Luk A, et al. Identification of a nuclear receptor for bile acids. Science. 1999; 284:1362–5.


4. Parks DJ, Blanchard SG, Bledsoe RK, Chandra G, Consler TG, Kliewer SA, et al. Bile acids: natural ligands for an orphan nuclear receptor. Science. 1999; 284:1365–8.


5. Maloney PR, Parks DJ, Haffner CD, Fivush AM, Chandra G, Plunket KD, et al. Identification of a chemical tool for the orphan nuclear receptor FXR. J Med Chem. 2000; 43:2971–4.


6. Cariou B, van Harmelen K, Duran-Sandoval D, van Dijk TH, Grefhorst A, Abdelkarim M, et al. The farnesoid X receptor modulates adiposity and peripheral insulin sensitivity in mice. J Biol Chem. 2006; 281:11039–49.


7. Lo Sasso G, Schlage WK, Boue S, Veljkovic E, Peitsch MC, Hoeng J. The Apoe(-/-) mouse model: a suitable model to study cardiovascular and respiratory diseases in the context of cigarette smoke exposure and harm reduction. J Transl Med. 2016; 14:146.


8. Sinal CJ, Tohkin M, Miyata M, Ward JM, Lambert G, Gonzalez FJ. Targeted disruption of the nuclear receptor FXR/BAR impairs bile acid and lipid homeostasis. Cell. 2000; 102:731–44.


9. Hanniman EA, Lambert G, McCarthy TC, Sinal CJ. Loss of functional farnesoid X receptor increases atherosclerotic lesions in apolipoprotein E-deficient mice. J Lipid Res. 2005; 46:2595–604.


10. Hartman HB, Gardell SJ, Petucci CJ, Wang S, Krueger JA, Evans MJ. Activation of farnesoid X receptor prevents atherosclerotic lesion formation in LDLR-/- and apoE-/- mice. J Lipid Res. 2009; 50:1090–100.


11. Miyazaki-Anzai S, Masuda M, Levi M, Keenan AL, Miyazaki M. Dual activation of the bile acid nuclear receptor FXR and G-protein-coupled receptor TGR5 protects mice against atherosclerosis. PLoS One. 2014; 9:e108270.


12. Armstrong LE, Guo GL. Role of FXR in liver inflammation during nonalcoholic steatohepatitis. Curr Pharmacol Rep. 2017; 3:92–100.


13. Sanyal AJ. Use of farnesoid X receptor agonists to treat nonalcoholic fatty liver disease. Dig Dis. 2015; 33:426–32.


14. Xu P, Zhai Y, Wang J. The role of PPAR and its cross-talk with CAR and LXR in obesity and atherosclerosis. Int J Mol Sci. 2018; 19:1260.


15. Kubota N, Terauchi Y, Kubota T, Kumagai H, Itoh S, Satoh H, et al. Pioglitazone ameliorates insulin resistance and diabetes by both adiponectin-dependent and -independent pathways. J Biol Chem. 2006; 281:8748–55.


16. Dasu MR, Park S, Devaraj S, Jialal I. Pioglitazone inhibits Toll-like receptor expression and activity in human monocytes and db/db mice. Endocrinology. 2009; 150:3457–64.


17. Ahn HY, Kim HH, Hwang JY, Park C, Cho BY, Park YJ. Effects of pioglitazone on nonalcoholic fatty liver disease in the absence of constitutive androstane receptor expression. PPAR Res. 2018; 2018:9568269.


18. Zhao W, Thacker SG, Hodgin JB, Zhang H, Wang JH, Park JL, et al. The peroxisome proliferator-activated receptor gamma agonist pioglitazone improves cardiometabolic risk and renal inflammation in murine lupus. J Immunol. 2009; 183:2729–40.


19. Gong Y, Shao Z, Fu Z, Edin ML, Sun Y, Liegl RG, et al. Fenofibrate inhibits cytochrome P450 epoxygenase 2C activity to suppress pathological ocular angiogenesis. EBioMedicine. 2016; 13:201–11.


20. Olivier P, Plancke MO, Marzin D, Clavey V, Sauzieres J, Fruchart JC. Effects of fenofibrate, gemfibrozil and nicotinic acid on plasma lipoprotein levels in normal and hyperlipidemic mice: a proposed model for drug screening. Atherosclerosis. 1988; 70:107–14.


21. Paraskevas KI, Pantopoulou A, Vlachos IS, Agrogiannis G, Iliopoulos DG, Karatzas G, et al. Comparison of fibrate, ezetimibe, low- and high-dose statin therapy for the dyslipidemia of the metabolic syndrome in a mouse model. Angiology. 2011; 62:144–54.


22. Kleiner DE, Brunt EM, Van Natta M, Behling C, Contos MJ, Cummings OW, et al. Design and validation of a histological scoring system for nonalcoholic fatty liver disease. Hepatology. 2005; 41:1313–21.


23. Watanabe M, Houten SM, Wang L, Moschetta A, Mangelsdorf DJ, Heyman RA, et al. Bile acids lower triglyceride levels via a pathway involving FXR, SHP, and SREBP-1c. J Clin Invest. 2004; 113:1408–18.


24. Shen LL, Liu H, Peng J, Gan L, Lu L, Zhang Q, et al. Effects of farnesoid X receptor on the expression of the fatty acid synthetase and hepatic lipase. Mol Biol Rep. 2011; 38:553–9.


25. Bugianesi E, McCullough AJ, Marchesini G. Insulin resistance: a metabolic pathway to chronic liver disease. Hepatology. 2005; 42:987–1000.


26. Andersson Y, Majd Z, Lefebvre AM, Martin G, Sechkin AV, Kosykh V, et al. Developmental and pharmacological regulation of apolipoprotein C-II gene expression: comparison with apo C-I and apo C-III gene regulation. Arterioscler Thromb Vasc Biol. 1999; 19:115–21.
27. Carlson LA, Ballantyne D. Changing relative proportions of apolipoproteins CII and CIII of very low density lipoproteins in hypertriglyceridaemia. Atherosclerosis. 1976; 23:563–8.


28. Erkelens DW, Brunzell JD, Bierman EL. Availability of apolipoprotein CII in relation to the maximal removal capacity for an infused triglyceride emulsion in man. Metabolism. 1979; 28:495–501.


29. Wang CS, McConathy WJ, Kloer HU, Alaupovic P. Modulation of lipoprotein lipase activity by apolipoproteins: effect of apolipoprotein C-III. J Clin Invest. 1985; 75:384–90.


30. Bishop-Bailey D. FXR as a novel therapeutic target for vascular disease. Drug News Perspect. 2004; 17:499–504.


31. Li J, Wilson A, Kuruba R, Zhang Q, Gao X, He F, et al. FXR-mediated regulation of eNOS expression in vascular endothelial cells. Cardiovasc Res. 2008; 77:169–77.


32. Mencarelli A, Cipriani S, Renga B, Francisci D, Palladino G, Distrutti E, et al. The bile acid sensor FXR protects against dyslipidemia and aortic plaques development induced by the HIV protease inhibitor ritonavir in mice. PLoS One. 2010; 5:e13238.


33. Miyazaki-Anzai S, Levi M, Kratzer A, Ting TC, Lewis LB, Miyazaki M. Farnesoid X receptor activation prevents the development of vascular calcification in ApoE-/- mice with chronic kidney disease. Circ Res. 2010; 106:1807–17.
34. Hambruch E, Miyazaki-Anzai S, Hahn U, Matysik S, Boettcher A, Perovic-Ottstadt S, et al. Synthetic farnesoid X receptor agonists induce high-density lipoprotein-mediated transhepatic cholesterol efflux in mice and monkeys and prevent atherosclerosis in cholesteryl ester transfer protein transgenic low-density lipoprotein receptor (-/-) mice. J Pharmacol Exp Ther. 2012; 343:556–67.


35. Liu HM, Lee TY, Liao JF. GW4064 attenuates lipopolysaccharide-induced hepatic inflammation and apoptosis through inhibition of the Toll-like receptor 4-mediated p38 mitogen-activated protein kinase signaling pathway in mice. Int J Mol Med. 2018; 41:1455–62.


36. Nevens F, Andreone P, Mazzella G, Strasser SI, Bowlus C, Invernizzi P, et al. A placebo-controlled trial of obeticholic acid in primary biliary cholangitis. N Engl J Med. 2016; 375:631–43.


37. Claudel T, Inoue Y, Barbier O, Duran-Sandoval D, Kosykh V, Fruchart J, et al. Farnesoid X receptor agonists suppress hepatic apolipoprotein CIII expression. Gastroenterology. 2003; 125:544–55.


38. Mencarelli A, Renga B, Distrutti E, Fiorucci S. Antiatherosclerotic effect of farnesoid X receptor. Am J Physiol Heart Circ Physiol. 2009; 296:H272–81.


39. Zhang Y, Wang X, Vales C, Lee FY, Lee H, Lusis AJ, et al. FXR deficiency causes reduced atherosclerosis in Ldlr-/- mice. Arterioscler Thromb Vasc Biol. 2006; 26:2316–21.
40. Pineda Torra I, Claudel T, Duval C, Kosykh V, Fruchart JC, Staels B. Bile acids induce the expression of the human peroxisome proliferator-activated receptor alpha gene via activation of the farnesoid X receptor. Mol Endocrinol. 2003; 17:259–72.
Fig. 1.
Atherosclerotic lesions of the aorta stained with Oil Red O in mice fed a Western diet (WD) with or without pioglitazone/fenofibrate treatment. (A) Representative photographs of aorta prepared using the en face method (a, apolipoprotein E [ApoE]−/− WD; b, ApoE−/− farnesoid X receptor [FXR]−/− WD; c, ApoE−/−FXR−/− WD+pioglitazone; d, ApoE−/−FXR−/− WD+fenofibrate). (B) Comparison of percentage of atherosclerotic lesions in the aorta in ApoE−/− (n=7) and ApoE−/−FXR−/− (n=9) mice fed a WD. The horizontal bars represent the mean percentage of atherosclerotic lesions. (C) Comparison of percentage of atherosclerotic lesions in the aorta in ApoE−/−FXR−/− mice fed a WD with or without pioglitazone (n=8) or fenofibrate (n=9) treatment. The horizontal bars represent the mean percentage of atherosclerotic lesions. Error bars show standard deviations. aA significant difference between ApoE−/− and ApoE−/−FXR−/− mice; bA significant difference between ApoE−/−FXR−/− mice with no treatment and ApoE−/−FXR−/− mice treated with fenofibrate.
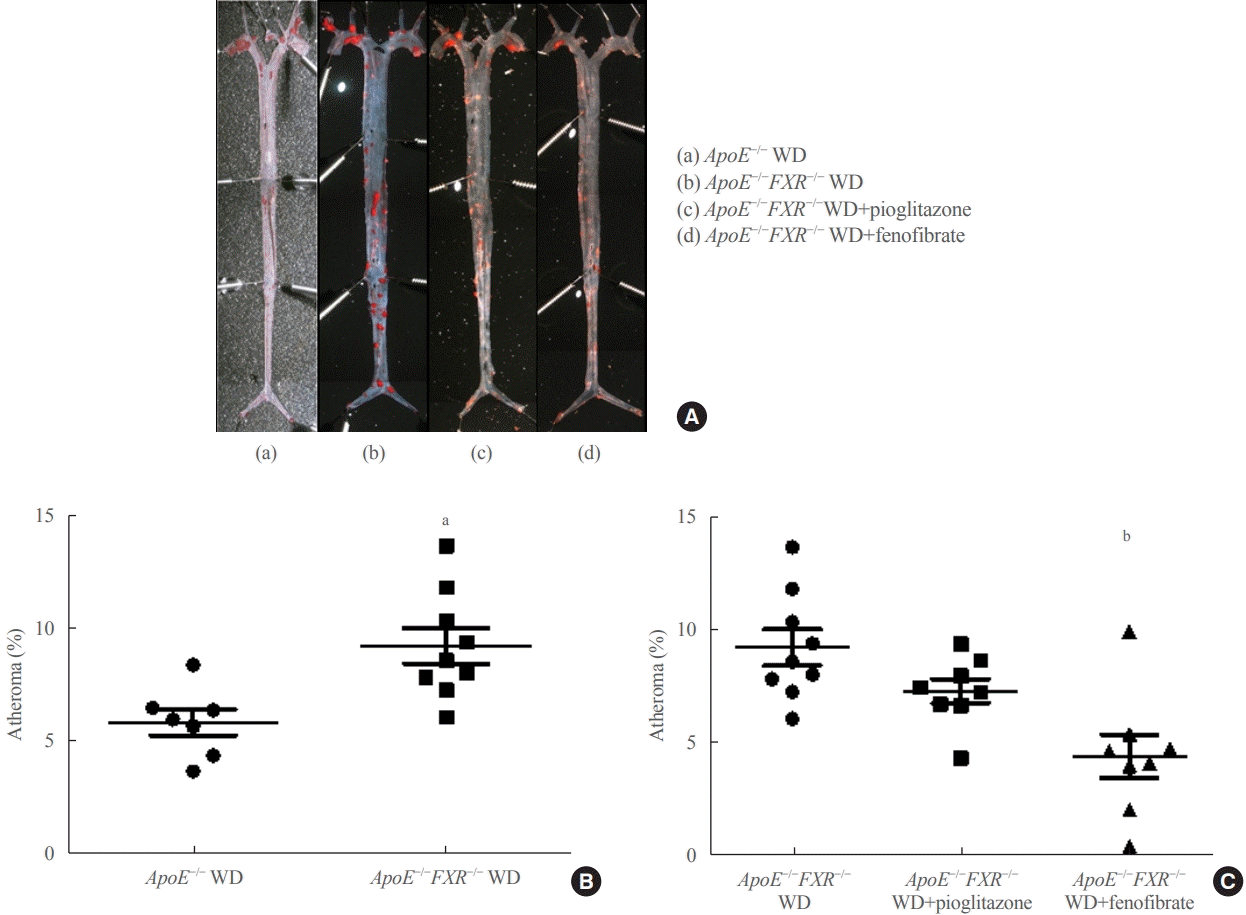
Fig. 2.
Comparison of liver histology and nonalcoholic fatty liver disease (NAFLD) activity scores (NAS) in apolipoprotein E (ApoE)−/− and ApoE−/− farnesoid X receptor (FXR)−/− mice fed a Western diet (WD) with or without pioglitazone or fenofibrate treatment. (A) Microscopic findings of the liver (H&E stain, ×200) (a, ApoE−/− [n=7]; b, ApoE−/−FXR−/− [n=9]; c, ApoE−/−FXR−/−+pioglitazone [n=8]; d, ApoE−/−FXR−/−+fenofibrate [n=9]). (B) Comparison of NAS between ApoE−/− (n=7) and ApoE−/−FXR−/− (n=9) mice. (C) Comparison of NAS among treatment groups in ApoE−/−FXR−/− mice (n=8 for control, n=8 for pioglitazone treatment, and n=9 for fenofibrate treatment). Error bars show standard deviations. aA significant difference between ApoE−/− and ApoE−/−FXR−/− mice.
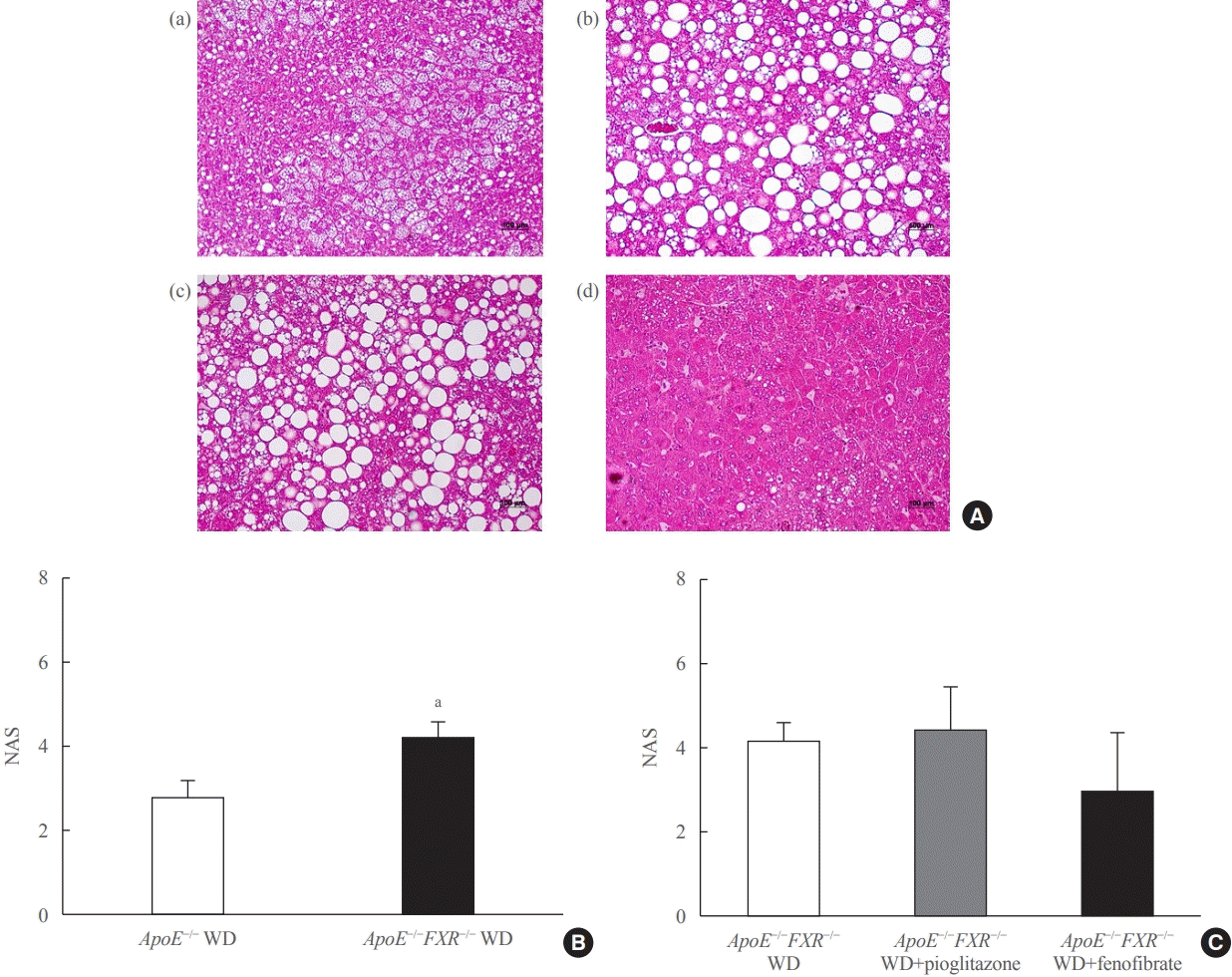
Fig. 3.
Comparison of gene expression related to lipid metabolism analyzed by quantitative polymerase chain reaction in liver, related to fatty acid synthesis (A), fatty acid uptake and catabolism (B), triglyceride hydrolysis (C), cholesterol metabolism (D), inflammation and hepatic fibrosis in the liver (E), lipolysis in adipose tissue (F), and mitochondrial activation in skeletal muscle (G) between apolipoprotein E (ApoE)−/− and ApoE−/− farnesoid X receptor (FXR)−/− mice. Error bars show standard deviations. WD, Western diet; SREBP1c, sterol regulatory element-binding protein 1c; FAS, fatty acid synthase; ACC1, acetyl-CoA carboxylase 1; SCD, stearoyl CoA desaturase; ACLY, ATP citrate lyase; FAT, fatty acid translocase; FATP1, fatty acid transport protein 1; CPT, carnitine palmitoyltranferase; ACS, acyl-CoA synthase; ACO, acyl-CoA oxidase; ACAA1A, acetyl-coenzyme A acyltransferease 1A; ApoC, apolipoprotein C; ANGPTL3, angiopoietin-like 3; PPAR, peroxisome proliferator-activated receptor; ApoA, apolipoprotein A; PLTP, phospholipid transfer protein; ABCG1, ATP-binding cassette sub-family G member 1; ABCA1, ATP-binding cassette transporter sub-family A member 1; SRB1, scavenger receptor class B type 1; CLA1, CD36 and LIMPII analogous-1; LXR, liver X receptor; MTP, microsomal triglyceride transfer protein; ApoB100, apolipoprotein B100; LDLR, low density lipoprotein receptor; TNFα, tumor necrosis factor-α; IL-6, interleukin-6; TGFβ, transforming growth factor β1; Col1α1, α1-collagen; TIMP, tissue inhibitor of metalloproteinase; αSMA, α smooth muscle actin; ATGL, adipocyte triglyceride lipase; TGH, triglycerol hydrolase; HSL, hormone sensitive lipase; MGL, monoglyceride lipase; Nrf-1, nuclear respiratory factor 1; PGC1, peroxisome proliferator-activated receptor gamma coactivator 1. aP<0.05.
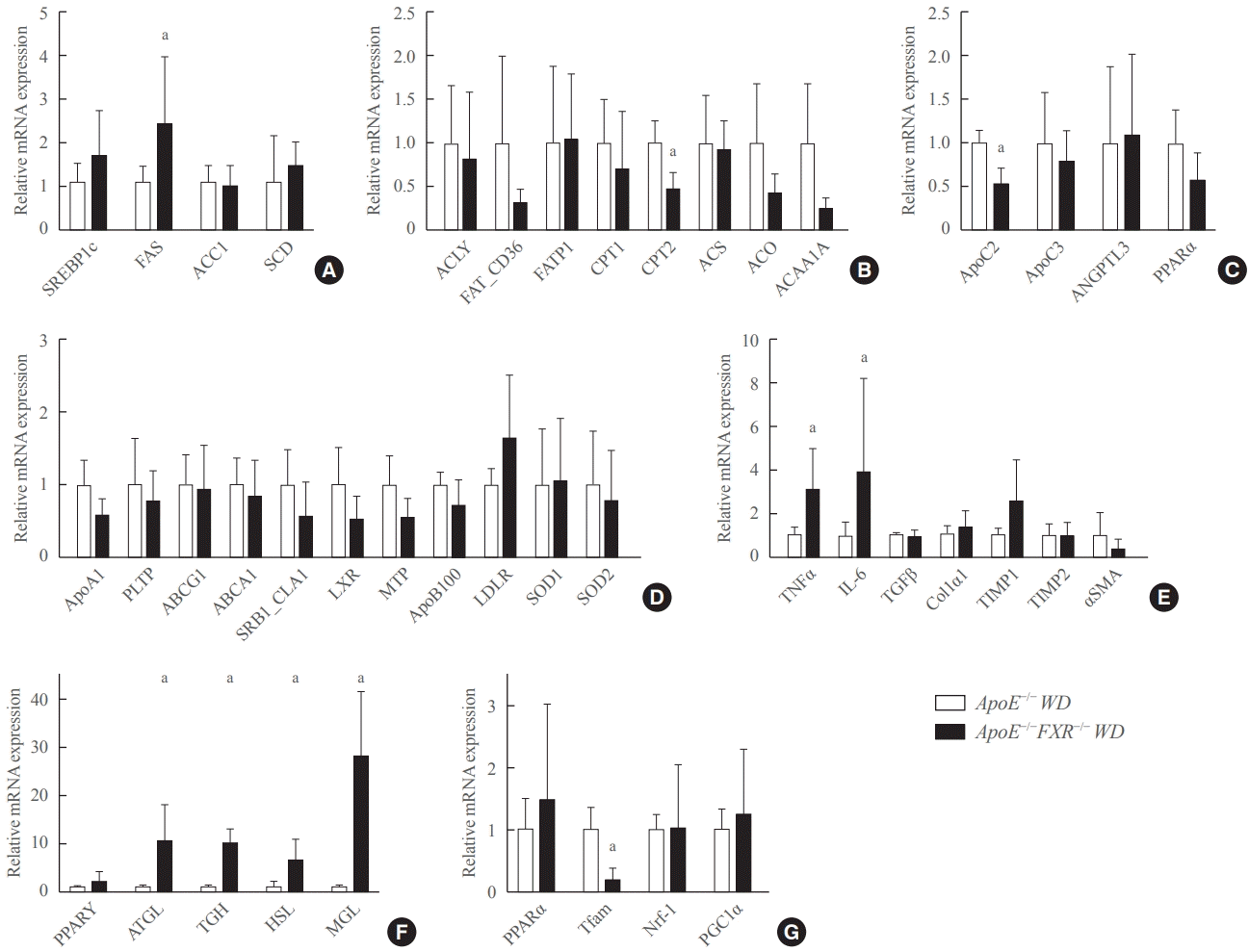
Fig. 4.
Comparison of gene expression related to lipid metabolism analyzed by quantitative polymerase chain reaction in the liver, related to fatty acid synthesis (A), fatty acid uptake and catabolism (B), triglyceride hydrolysis (C), cholesterol metabolism (D), inflammation and hepatic fibrosis in the liver (E), lipolysis in adipose tissue (F), and mitochondrial activation in skeletal muscle (G) among apolipoprotein E (ApoE)−/− farnesoid X receptor (FXR)−/− mice with or without fenofibrate or pioglitazone treatment. Error bars show standard deviations. WD, Western diet; SREBP1c, sterol regulatory element-binding protein 1c; FAS, fatty acid synthase; ACC1, acetyl-CoA carboxylase 1; SCD, stearoyl CoA desaturase; ACLY, ATP citrate lyase; FAT, fatty acid translocase; FATP1, fatty acid transport protein 1; CPT, carnitine palmitoyltranferase; ACS, acyl-CoA synthase; ACO, acyl-CoA oxidase; ACAA1A, acetyl-coenzyme A acyltransferease 1A; ApoC, apolipoprotein C; ANGPTL3, angiopoietin-like 3; PPAR, peroxisome proliferator-activated receptor; ApoA, apolipoprotein A; PLTP, phospholipid transfer protein; ABCG1, ATP-binding cassette sub-family G member 1; ABCA1, ATP-binding cassette transporter sub-family A member 1; SRB1, scavenger receptor class B type 1; CLA1, CD36 and LIMPII analogous-1; LXR, liver X receptor; MTP, microsomal triglyceride transfer protein; ApoB100, apolipoprotein B100; LDLR, low density lipoprotein receptor; SOD, superoxide dismutase; TNFα, tumor necrosis factor-α; IL-6, interleukin-6; TGFβ, transforming growth factor β1; Col1α1, α1-collagen; TIMP, tissue inhibitor of metalloproteinase; αSMA, α smooth muscle actin; ATGL, adipocyte triglyceride lipase; TGH, triglycerol hydrolase; HSL, hormone sensitive lipase; MGL, monoglyceride lipase; Nrf-1, nuclear respiratory factor 1; PGC1, peroxisome proliferator-activated receptor gamma coactivator 1. aP<0.05.
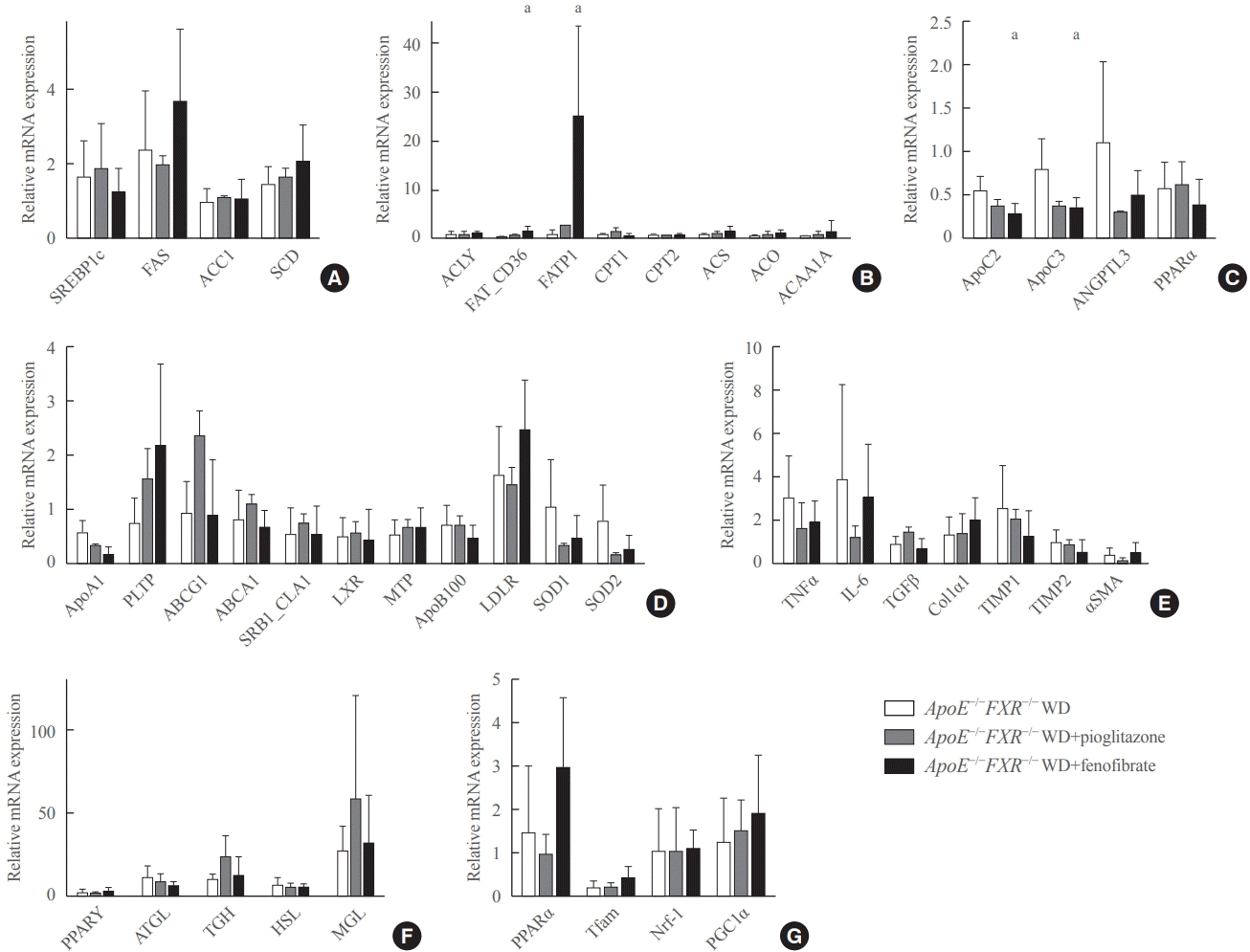