Abstract
Skeletal muscle has attracted attention as endocrine organ, because exercise-dependent cytokines called myokines/exerkines are released from skeletal muscle and are involved in systemic functions. While, local mechanical loading to skeletal muscle by exercise or resistance training alters myofiber type and size and myonuclear number. Skeletal muscle-resident stem cells, known as muscle satellite cells (MuSCs), are responsible for the increased number of myonuclei. Under steady conditions, MuSCs are maintained in a mitotically quiescent state but exit from that state and start to proliferate in response to high physical activity. Alterations in MuSC behavior occur when myofibers are damaged, but the lethal damage to myofibers does not seem to evoke mechanical loading-dependent MuSC activation and proliferation. Given that MuSCs proliferate without damage, it is unclear how the different behaviors of MuSCs are controlled by different physical activities. Recent studies demonstrated that myonuclear number reflects the size of myofibers; hence, it is crucial to know the properties of MuSCs and the mechanism of myonuclear accretion by MuSCs. In addition, the elucidation of mechanical load-dependent changes in muscle resident cells, including MuSCs, will be necessary for the discovery of new myokines/exerkines and understating skeletal muscle diseases.
Physical activity is defined as any bodily movement produced by skeletal muscles that requires energy expenditure [1]. Exercise is a subset of physical activity that is planned, structured, and repetitive [1] and exerts many beneficial effects on the body across species [2,3]. Exercise is a key factor in the prevention and treatment of metabolic diseases. It has also been demonstrated that exercise provides protection from stress-induced depression via induction of the expression of kynurenine aminotransferases [4]. In addition, exercise exerts anti-tumor effects [5,6]. These beneficial effects are considered to be provided by myokines, also called exerkines, that are released from multinuclear cells, myofibers, composing skeletal muscle [4,5]. Recent studies of potential exerkines or exercise-dependent factors are briefly summarized in Table 1 [4–10]. Myofiber itself, including the component of myosin heavy chain isoform, extracellular remodeling, and the number of nuclei in myofibers are affected by exercise [11]. As the amount of circulating exerkines is limited, for identifying new exerkines, it is critical to know the cellular network and the direct effects of mechanical cues on skeletal muscle loaded by exercise. In addition to myofibers, skeletal muscle contains muscle satellite cells (MuSCs), endothelial cells, and mesenchymal progenitor cells (also called fibro/adipogenic progenitors [FAPs]) [12]; MuSCs play critical roles in muscle hypertrophy induced by mechanical loading. Here, we review the behaviors of MuSCs in skeletal muscle loaded by exercise or resistance training in mouse models.
The muscle stem cell is the defined as a mononuclear cell located in a unique position. In 1961, Dr. Mauro [13] found mononuclear cells located between the basal lamina and sarcolemma (cell membrane of myofiber) in frog muscle and named them ‘satellite cells.’ As predicted by Dr. Mauro, MuSCs are indispensable for skeletal muscle regeneration [14,15]. Pax7, M-cadherin, integrin α7, and Vcam1 are widely used for the identification and purification of MuSC [16–20]. We identified calcitonin receptor (CalcR) as a quiescent MuSC-specific cell surface molecule in both humans and mice [21–23].
MuSCs are heterogeneous populations with different cell cycling times [24,25], Pax3 [26], Pax7 [27], Myf5 [28], Mx1 [29], or CD34 expression [30]. In addition, MuSC properties are also influenced by myofiber type [31] or the muscle region in the body [32]. MuSCs in both slow (soleus) and fast-type muscles (plantaris) can supply new myonuclei in loading muscles [33,34], suggesting that the responsiveness of MuSCs to mechanical loading is not dramatically different between slow and fast myofibers. However, the correlation between heterogeneity in marker expression and behavior of MuSCs in loaded muscles has not been demonstrated.
Initially, it was thought that MuSCs had multi-differentiation potential involving mainly mesenchymal lineage: adipocytes, osteocytes, and fibroblastic cells. Even when adult MuSCs lose both myogenic differentiation 1 (MyoD) and myogenic factor 5 (Myf5) that are essential myogenic determination factors, the MuSC-pool is sustained in uninjured muscle [35]. However, proliferating MuSCs lose their myogenic identity; alternatively, they differentiate into adipogenic and fibroblastic cells [35]. These results indicate that MyoD and Myf5 are essential for the myogenic identity of adult proliferating MuSCs. Whereas, MuSC is not the main cell source of adipogenic and fibroblastic cells even in disease and aged conditions. In skeletal muscle, mesenchymal progenitors (also called FAPs) mainly provide such types of cells in disease conditions [36–38], and the contributions of MuSCs to non-myogenic lineage cells are rare. It has been demonstrated that MuSCs are the only cells that provide new myonuclei in skeletal muscles loaded by running exercise or resistance training in mouse models [39,40].
It is of note that MuSCs are directly attached to myofibers that randomly contract and relax even in a sedentary condition, where MuSCs can maintain quiescence. However, intense contractions induce MuSC activation and proliferation. Given that the muscle regeneration process is not involved in MuSCs activation and proliferation in loaded muscles [41–43], these results indicate that MuSCs responses are strongly correlated with physical activity and strength of myofiber contraction (Fig. 1). Since several signaling pathways actively sustain MuSC quiescence even in sedentary mice [44], the reduction in these pathways might be the initiation of MuSC activation in loaded muscle. CalcR is a potent candidate because of its role and specific expression in quiescent MuSCs [22,45,46]. We have investigated the role of CalcR in physically active mice. It could also be expected that the exercise or resistance training models would allow us to find new aspects of maintenance signaling in MuSCs.
MyoD suppression is regulated by canonical Notch signaling which is also fundamentally important for MuSC maintenance [47,48]. The hairy and enhancer of split (Hes)-Hey (Hes-related, also known as Hesr/Herp/Hrt/Gridlock/Chf) heterodimer is involved in a predicted mechanism as the downstream of Notch signaling for suppressing MyoD expression [49,50]. The loss of Notch-related genes in adult MuSCs leads to MyoD expression, and MuSCs eventually fuse to existing myofibers [49,51–53]. In regenerating muscles or in vitro culture conditions, a rapid increase in MyoD protein level is a hallmark of MuSC activation before cell cycle entry, and then MuSCs proliferate with MyoD expression (Fig. 2) [54]. However, we found that majority of proliferating MuSCs do not express MyoD in a mouse resistance training model (Fig. 2) [42]. We also found that HeyL expression was maintained at a high level in proliferating MuSCs in the overloaded model. Although HeyL-deficient mice did not show a pronounced phenotype in muscle regeneration models, in the overloaded model, they exhibited blunted muscle hypertrophy due to the reduced number of new myonuclei. As observed in Notch signaling mutant mice, MyoD expression allows MuSCs to fuse with myofibers, suggesting that the suppression of MyoD expression in proliferating MuSCs is essential to inhibit their incorporation into myofibers during their proliferation on myofibers.
The reduced number and regenerative ability of MuSCs during aging are well-documented in mouse models [44]. Blunted muscle hypertrophy in aged mice was also reported in a surgical resistance training model, although myonuclear accretion had occurred [55]. In addition to intrinsic impairments in aged MuSCs, the environment for muscle regeneration is disrupted by aging [56,57]. In contrast, questions such as whether MuSCs are responsive to mechanical loading and whether the environment inducing MuSC expansion in aged skeletal muscle changes remain to be explored. Of note, the beneficial effects of exercise on muscle regeneration or rejuvenation of aged MuSCs have been reported [58,59]. If the behavior of aged MuSCs in response to exercise is comparable to that of young MuSCs, it is possible that exercise improves the behavior of aged MuSCs in loading muscles. Analyses of aged MuSCs behaviors in response to exercise/resistance training remain to be conducted. It is also interesting to explore the quality of new myonuclei supplied by aged MuSCs compared with those supplied by young MuSCs in exercise/resistance training muscles.
A characteristic feature of muscle hypertrophy induced by mechanical loading is an increase in myofiber size, thus requiring an increase in protein synthesis. Another feature is an increase in the number of myonuclei. Since there is a limit to the cytoplasmic area that can be dominated by a single nucleus of a myofiber, new myonuclei must be generated to maintain a constant cytoplasmic area; this is known as the myonuclear domain theory. It is also considered that the number of myonuclei must increase to supply ribosomes that are the rate-limiting factor for protein synthesis. Since only MuSCs can supply nuclei to myofibers, MuSCs are generally considered essential for muscle hypertrophy. However, the debate on whether MuSC was necessary for hypertrophy occurred in 2007 [60]. At that time, methodological issues remained. Ten years later, the same debate recurred with two different reports using genetically modified MuSC-depleted mice [61,62]. As O’Connor and Pavlath [60] stated, short-term hypertrophy may not require an increase in the number of myonuclei, but no conclusion can be reached without a long-term experiment. To the best of our knowledge, all research groups observed blunted muscle hypertrophy at least in long-term experiments when myonuclear accretion was impaired [42,63]. Accumulating evidence also suggests the critical role of myonuclear accretion by MuSCs in both exercise and resistance training animal models [64–67]. Thus, it can be concluded that MuSCs are necessary for muscle hypertrophy induced by mechanical loading [41].
A recent study has shown that, in developing myofibers, there is some tolerance to the transcriptional capacity of myonuclei. In myofibers with approximately half of the nuclei, the transcriptional capacity of total RNA in one nucleus is doubled [68]. However, interestingly, the reserve capacity is reduced in myofibers harboring 75% of myonuclei; thus, the myofiber size was similarly reduced to that of the myofibers with half the nuclei [68]. It has also been demonstrated that the number of myonuclei defines the size of myofibers in both humans and mice [69]. These studies also indicate the critical roles of myonuclear number and MuSCs, because MuSC is also responsible for the increase in myonuclear number during the developmental stage [70].
In a muscle mechanically loaded by exercise or resistance training, MuSCs start to proliferate and eventually supply new myonuclei that are critical for efficient muscle hypertrophy. MuSCs in loaded muscles proliferate by mechanisms different from those in regenerating muscle, one of which is a sustained expression of HeyL. As myonuclei number, and perhaps also the quality, directly influence the myofiber size, MuSC-mediated control of these parameters might be an innovative therapeutic approach for muscular disorders, including sarcopenia. Elucidation of the molecular mechanisms underlying the MuSC activation and proliferation and the related cellular network will lead to the discovery of new aspects of skeletal muscles as an endocrine organ.
ACKNOWLEDGMENTS
This study was funded by a Grant-in-Aid for Scientific Research (B, 19H04000), Challenging Research (Exploratory, 20K21757), and an Intramural Research Grant for Neurological and Psychiatric Disorders of National Center of Neurology and Psychiatry (NCNP) (2–6). We also thank Akihiro Kaneshige and Sumiaki Fukuda for providing pictures of MuSCs shown in Fig. 2.
REFERENCES
1. Caspersen CJ, Powell KE, Christenson GM. Physical activity, exercise, and physical fitness: definitions and distinctions for health-related research. Public Health Rep. 1985; 100:126–31.
2. Hartman JH, Smith LL, Gordon KL, Laranjeiro R, Driscoll M, Sherwood DR, et al. Swimming exercise and transient food deprivation in caenorhabditis elegans promote mitochondrial maintenance and protect against chemical-induced mitotoxicity. Sci Rep. 2018; 8:8359.


3. Cartee GD, Hepple RT, Bamman MM, Zierath JR. Exercise promotes healthy aging of skeletal muscle. Cell Metab. 2016; 23:1034–47.


4. Agudelo LZ, Femenia T, Orhan F, Porsmyr-Palmertz M, Goiny M, Martinez-Redondo V, et al. Skeletal muscle PGC-1α1 modulates kynurenine metabolism and mediates resilience to stress-induced depression. Cell. 2014; 159:33–45.


5. Pedersen L, Idorn M, Olofsson GH, Lauenborg B, Nookaew I, Hansen RH, et al. Voluntary running suppresses tumor growth through epinephrine- and IL-6-dependent NK cell mobilization and redistribution. Cell Metab. 2016; 23:554–62.


6. Aoi W, Naito Y, Takagi T, Tanimura Y, Takanami Y, Kawai Y, et al. A novel myokine, secreted protein acidic and rich in cysteine (SPARC), suppresses colon tumorigenesis via regular exercise. Gut. 2013; 62:882–9.


7. Vinel C, Lukjanenko L, Batut A, Deleruyelle S, Pradere JP, Le Gonidec S, et al. The exerkine apelin reverses age-associated sarcopenia. Nat Med. 2018; 24:1360–71.


8. Son JS, Zhao L, Chen Y, Chen K, Chae SA, de Avila JM, et al. Maternal exercise via exerkine apelin enhances brown adipogenesis and prevents metabolic dysfunction in offspring mice. Sci Adv. 2020; 6:eaaz0359.


9. Laurens C, Parmar A, Murphy E, Carper D, Lair B, Maes P, et al. Growth and differentiation factor 15 is secreted by skeletal muscle during exercise and promotes lipolysis in humans. JCI Insight. 2020; 5:e131870.


10. Reddy A, Bozi LHM, Yaghi OK, Mills EL, Xiao H, Nicholson HE, et al. pH-gated succinate secretion regulates muscle remodeling in response to exercise. Cell. 2020; 183:62–75.


11. Bamman MM, Roberts BM, Adams GR. Molecular regulation of exercise-induced muscle fiber hypertrophy. Cold Spring Harb Perspect Med. 2018; 8:a029751.


12. Evano B, Tajbakhsh S. Skeletal muscle stem cells in comfort and stress. NPJ Regen Med. 2018; 3:24.


14. Lepper C, Partridge TA, Fan CM. An absolute requirement for Pax7-positive satellite cells in acute injury-induced skeletal muscle regeneration. Development. 2011; 138:3639–46.


15. Sambasivan R, Yao R, Kissenpfennig A, Van Wittenberghe L, Paldi A, Gayraud-Morel B, et al. Pax7-expressing satellite cells are indispensable for adult skeletal muscle regeneration. Development. 2011; 138:3647–56.


16. Seale P, Sabourin LA, Girgis-Gabardo A, Mansouri A, Gruss P, Rudnicki MA. Pax7 is required for the specification of myogenic satellite cells. Cell. 2000; 102:777–86.


17. Irintchev A, Zeschnigk M, Starzinski-Powitz A, Wernig A. Expression pattern of M-cadherin in normal, denervated, and regenerating mouse muscles. Dev Dyn. 1994; 199:326–37.


18. Rosen GD, Sanes JR, LaChance R, Cunningham JM, Roman J, Dean DC. Roles for the integrin VLA-4 and its counter receptor VCAM-1 in myogenesis. Cell. 1992; 69:1107–19.


19. Blanco-Bose WE, Yao CC, Kramer RH, Blau HM. Purification of mouse primary myoblasts based on alpha 7 integrin expression. Exp Cell Res. 2001; 265:212–20.
20. Fukada S, Ma Y, Ohtani T, Watanabe Y, Murakami S, Yamaguchi M. Isolation, characterization, and molecular regulation of muscle stem cells. Front Physiol. 2013; 4:317.


21. Ikemoto-Uezumi M, Uezumi A, Zhang L, Zhou H, Hashimoto N, Okamura K, et al. Reduced expression of calcitonin receptor is closely associated with age-related loss of the muscle stem cell pool. JCSM Rapid Commun. 2019; 2:1–13.


22. Fukada S, Uezumi A, Ikemoto M, Masuda S, Segawa M, Tanimura N, et al. Molecular signature of quiescent satellite cells in adult skeletal muscle. Stem Cells. 2007; 25:2448–59.


23. Yamaguchi M, Ogawa R, Watanabe Y, Uezumi A, Miyagoe-Suzuki Y, Tsujikawa K, et al. Calcitonin receptor and Odz4 are differently expressed in Pax7-positive cells during skeletal muscle regeneration. J Mol Histol. 2012; 43:581–7.


24. Chakkalakal JV, Jones KM, Basson MA, Brack AS. The aged niche disrupts muscle stem cell quiescence. Nature. 2012; 490:355–60.


25. Ono Y, Masuda S, Nam HS, Benezra R, Miyagoe-Suzuki Y, Takeda S. Slow-dividing satellite cells retain long-term self-renewal ability in adult muscle. J Cell Sci. 2012; 125(Pt 5):1309–17.


26. Der Vartanian A, Quetin M, Michineau S, Aurade F, Hayashi S, Dubois C, et al. PAX3 confers functional heterogeneity in skeletal muscle stem cell responses to environmental stress. Cell Stem Cell. 2019; 24:958–73.


27. Rocheteau P, Gayraud-Morel B, Siegl-Cachedenier I, Blasco MA, Tajbakhsh S. A subpopulation of adult skeletal muscle stem cells retains all template DNA strands after cell division. Cell. 2012; 148:112–25.


28. Kuang S, Kuroda K, Le Grand F, Rudnicki MA. Asymmetric self-renewal and commitment of satellite stem cells in muscle. Cell. 2007; 129:999–1010.


29. Scaramozza A, Park D, Kollu S, Beerman I, Sun X, Rossi DJ, et al. Lineage tracing reveals a subset of reserve muscle stem cells capable of clonal expansion under stress. Cell Stem Cell. 2019; 24:944–57.


30. Garcia-Prat L, Perdiguero E, Alonso-Martin S, Dell’Orso S, Ravichandran S, Brooks SR, et al. FoxO maintains a genuine muscle stem-cell quiescent state until geriatric age. Nat Cell Biol. 2020; 22:1307–18.


31. Motohashi N, Uezumi A, Asakura A, Ikemoto-Uezumi M, Mori S, Mizunoe Y, et al. Tbx1 regulates inherited metabolic and myogenic abilities of progenitor cells derived from slow- and fast-type muscle. Cell Death Differ. 2019; 26:1024–36.


32. Evano B, Gill D, Hernando-Herraez I, Comai G, Stubbs TM, Commere PH, et al. Transcriptome and epigenome diversity and plasticity of muscle stem cells following transplantation. PLoS Genet. 2020; 16:e1009022.


33. Li P, Akimoto T, Zhang M, Williams RS, Yan Z. Resident stem cells are not required for exercise-induced fiber-type switching and angiogenesis but are necessary for activity-dependent muscle growth. Am J Physiol Cell Physiol. 2006; 290:C1461–8.


34. Masschelein E, D’Hulst G, Zvick J, Hinte L, Soro-Arnaiz I, Gorski T, et al. Exercise promotes satellite cell contribution to myofibers in a load-dependent manner. Skelet Muscle. 2020; 10:21.


35. Yamamoto M, Legendre NP, Biswas AA, Lawton A, Yamamoto S, Tajbakhsh S, et al. Loss of MyoD and Myf5 in skeletal muscle stem cells results in altered myogenic programming and failed regeneration. Stem Cell Reports. 2018; 10:956–69.


36. Uezumi A, Fukada S, Yamamoto N, Takeda S, Tsuchida K. Mesenchymal progenitors distinct from satellite cells contribute to ectopic fat cell formation in skeletal muscle. Nat Cell Biol. 2010; 12:143–52.


37. Joe AW, Yi L, Natarajan A, Le Grand F, So L, Wang J, et al. Muscle injury activates resident fibro/adipogenic progenitors that facilitate myogenesis. Nat Cell Biol. 2010; 12:153–63.


38. Uezumi A, Ito T, Morikawa D, Shimizu N, Yoneda T, Segawa M, et al. Fibrosis and adipogenesis originate from a common mesenchymal progenitor in skeletal muscle. J Cell Sci. 2011; 124(Pt 21):3654–64.


39. McCarthy JJ, Mula J, Miyazaki M, Erfani R, Garrison K, Farooqui AB, et al. Effective fiber hypertrophy in satellite cell-depleted skeletal muscle. Development. 2011; 138:3657–66.


40. Englund DA, Murach KA, Dungan CM, Figueiredo VC, Vechetti IJ Jr, Dupont-Versteegden EE, et al. Depletion of resident muscle stem cells negatively impacts running volume, physical function, and muscle fiber hypertrophy in response to lifelong physical activity. Am J Physiol Cell Physiol. 2020; 318:C1178–88.


41. Fukada SI, Akimoto T, Sotiropoulos A. Role of damage and management in muscle hypertrophy: different behaviors of muscle stem cells in regeneration and hypertrophy. Biochim Biophys Acta Mol Cell Res. 2020; 1867:118742.


42. Fukuda S, Kaneshige A, Kaji T, Noguchi YT, Takemoto Y, Zhang L, et al. Sustained expression of HeyL is critical for the proliferation of muscle stem cells in overloaded muscle. Elife. 2019; 8:e48284.


43. Darr KC, Schultz E. Exercise-induced satellite cell activation in growing and mature skeletal muscle. J Appl Physiol (1985). 1987; 63:1816–21.


44. Fuchs E, Blau HM. Tissue stem cells: architects of their niches. Cell Stem Cell. 2020; 27:532–56.


45. Zhang L, Noguchi YT, Nakayama H, Kaji T, Tsujikawa K, Ikemoto-Uezumi M, et al. The CalcR-PKA-Yap1 axis is critical for maintaining quiescence in muscle stem cells. Cell Rep. 2019; 29:2154–63.


46. Yamaguchi M, Watanabe Y, Ohtani T, Uezumi A, Mikami N, Nakamura M, et al. Calcitonin receptor signaling inhibits muscle stem cells from escaping the quiescent state and the niche. Cell Rep. 2015; 13:302–14.


47. Bjornson CR, Cheung TH, Liu L, Tripathi PV, Steeper KM, Rando TA. Notch signaling is necessary to maintain quiescence in adult muscle stem cells. Stem Cells. 2012; 30:232–42.


48. Mourikis P, Sambasivan R, Castel D, Rocheteau P, Bizzarro V, Tajbakhsh S. A critical requirement for notch signaling in maintenance of the quiescent skeletal muscle stem cell state. Stem Cells. 2012; 30:243–52.


49. Noguchi YT, Nakamura M, Hino N, Nogami J, Tsuji S, Sato T, et al. Cell-autonomous and redundant roles of Hey1 and HeyL in muscle stem cells: HeyL requires Hes1 to bind diverse DNA sites. Development. 2019; 146:dev163618.


50. Lahmann I, Brohl D, Zyrianova T, Isomura A, Czajkowski MT, Kapoor V, et al. Oscillations of MyoD and Hes1 proteins regulate the maintenance of activated muscle stem cells. Genes Dev. 2019; 33:524–35.


51. Fukada S, Yamaguchi M, Kokubo H, Ogawa R, Uezumi A, Yoneda T, et al. Hesr1 and Hesr3 are essential to generate undifferentiated quiescent satellite cells and to maintain satellite cell numbers. Development. 2011; 138:4609–19.


52. Fujimaki S, Seko D, Kitajima Y, Yoshioka K, Tsuchiya Y, Masuda S, et al. Notch1 and notch2 coordinately regulate stem cell function in the quiescent and activated states of muscle satellite cells. Stem Cells. 2018; 36:278–85.


53. Mizuno S, Yoda M, Shimoda M, Tohmonda T, Okada Y, Toyama Y, et al. A disintegrin and metalloprotease 10 (ADAM10) is indispensable for maintenance of the muscle satellite cell pool. J Biol Chem. 2015; 290:28456–64.


54. Zammit PS, Golding JP, Nagata Y, Hudon V, Partridge TA, Beauchamp JR. Muscle satellite cells adopt divergent fates: a mechanism for self-renewal? J Cell Biol. 2004; 166:347–57.
55. Lee JD, Fry CS, Mula J, Kirby TJ, Jackson JR, Liu F, et al. Aged muscle demonstrates fiber-type adaptations in response to mechanical overload, in the absence of myofiber hypertrophy, independent of satellite cell abundance. J Gerontol A Biol Sci Med Sci. 2016; 71:461–7.


56. Ikemoto-Uezumi M, Uezumi A, Tsuchida K, Fukada S, Yamamoto H, Yamamoto N, et al. Pro-insulin-like growth factor-II ameliorates age-related inefficient regenerative response by orchestrating self-reinforcement mechanism of muscle regeneration. Stem Cells. 2015; 33:2456–68.


57. Lukjanenko L, Karaz S, Stuelsatz P, Gurriaran-Rodriguez U, Michaud J, Dammone G, et al. Aging disrupts muscle stem cell function by impairing matricellular WISP1 secretion from fibro-adipogenic progenitors. Cell Stem Cell. 2019; 24:433–46.


58. Brett JO, Arjona M, Ikeda M, Quarta M, de Morree A, Egner IM, et al. Exercise rejuvenates quiescent skeletal muscle stem cells in old mice through restoration of cyclin D1. Nat Metab. 2020; 2:307–17.


59. Joanisse S, Nederveen JP, Baker JM, Snijders T, Iacono C, Parise G. Exercise conditioning in old mice improves skeletal muscle regeneration. FASEB J. 2016; 30:3256–68.


60. O’Connor RS, Pavlath GK. Point: counterpoint: satellite cell addition is/is not obligatory for skeletal muscle hypertrophy. J Appl Physiol (1985). 2007; 103:1099–100.
61. McCarthy JJ, Dupont-Versteegden EE, Fry CS, Murach KA, Peterson CA. Methodological issues limit interpretation of negative effects of satellite cell depletion on adult muscle hypertrophy. Development. 2017; 144:1363–5.


62. Egner IM, Bruusgaard JC, Gundersen K. Satellite cell depletion prevents fiber hypertrophy in skeletal muscle. Development. 2016; 143:2898–906.


63. Fry CS, Lee JD, Jackson JR, Kirby TJ, Stasko SA, Liu H, et al. Regulation of the muscle fiber microenvironment by activated satellite cells during hypertrophy. FASEB J. 2014; 28:1654–65.
64. Goh Q, Millay DP. Requirement of myomaker-mediated stem cell fusion for skeletal muscle hypertrophy. Elife. 2017; 6:e20007.


65. Goh Q, Song T, Petrany MJ, Cramer AA, Sun C, Sadayappan S, et al. Myonuclear accretion is a determinant of exercise-induced remodeling in skeletal muscle. Elife. 2019; 8:e44876.


66. Moriya N, Miyazaki M. Akt1 deficiency diminishes skeletal muscle hypertrophy by reducing satellite cell proliferation. Am J Physiol Regul Integr Comp Physiol. 2018; 314:R741–51.


67. Randrianarison-Huetz V, Papaefthymiou A, Herledan G, Noviello C, Faradova U, Collard L, et al. Srf controls satellite cell fusion through the maintenance of actin architecture. J Cell Biol. 2018; 217:685–700.


68. Cramer AAW, Prasad V, Eftestol E, Song T, Hansson KA, Dugdale HF, et al. Nuclear numbers in syncytial muscle fibers promote size but limit the development of larger myonuclear domains. Nat Commun. 2020; 11:6287.


Fig. 1
Behaviors of muscle stem cells during different physical activities. (A) During sedentary or light physical activity, muscle satellite cells (MuSCs) remain in a quiescent state. (B) During intense physical activity, MuSCs start to proliferate.
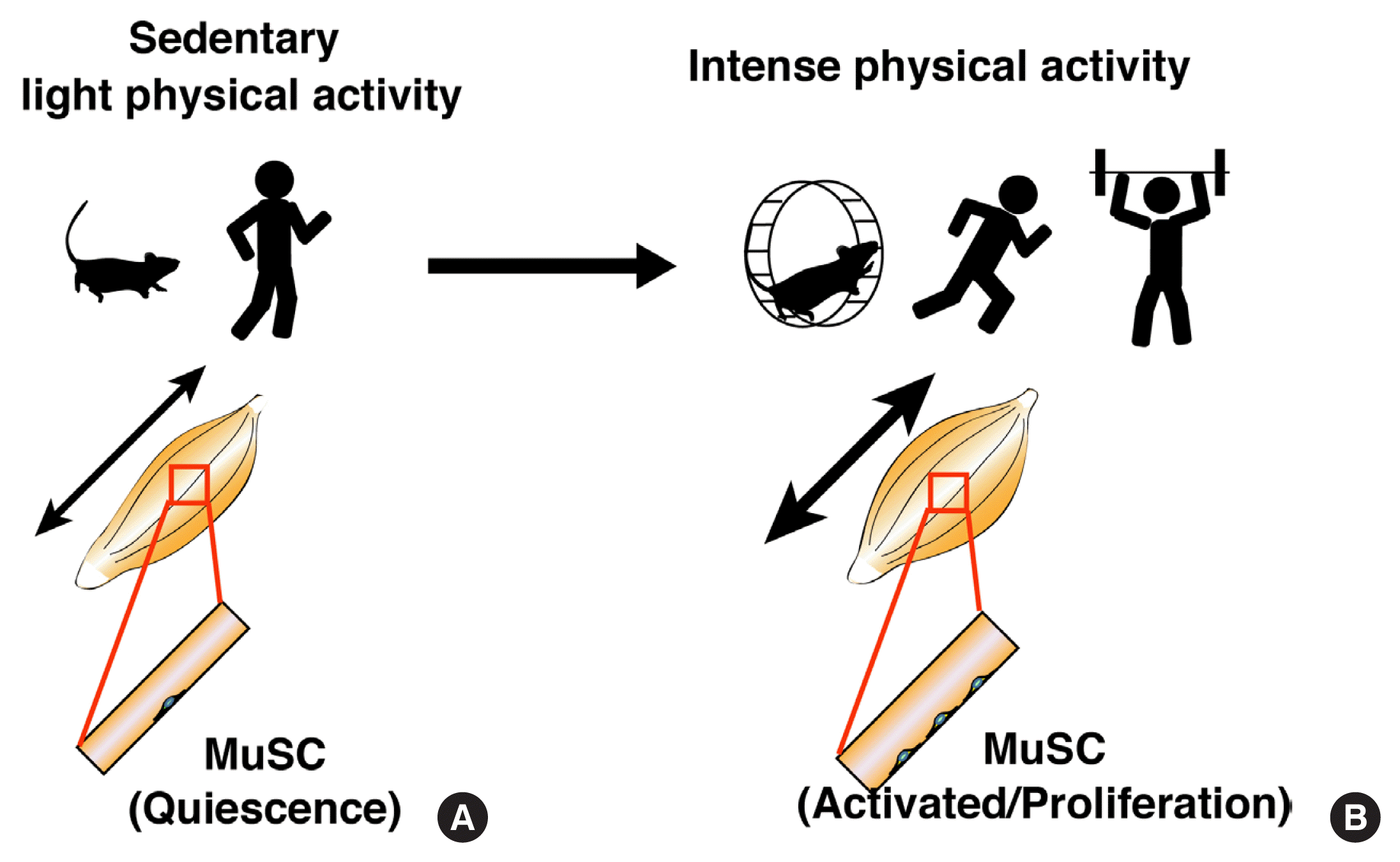
Fig. 2
Muscle stem cells on myofibers. (A) Freshly isolated myofibers were stained with an anti-Pax7 antibody (green). Arrows indicate Pax7-positive muscle satellite cells (MuSCs). In this Figure, cartoon shows the expression of Hey and myogenic differentiation (MyoD) in MuSCs under each condition. (B) Cultured myofibers were stained with anti-Pax7 (red), MyoD (green), and Ki67 (cyan) antibodies. Three days after culturing in vitro, MuSCs expressed MyoD and Ki67. (C) Freshly isolated myofibers from overloaded muscles were stained with anti-Pax7 (red), MyoD (green), and Ki67 (white) antibodies. MuSCs proliferate without MyoD expression. DAPI, 4′,6-diamidino-2-phenylindole.
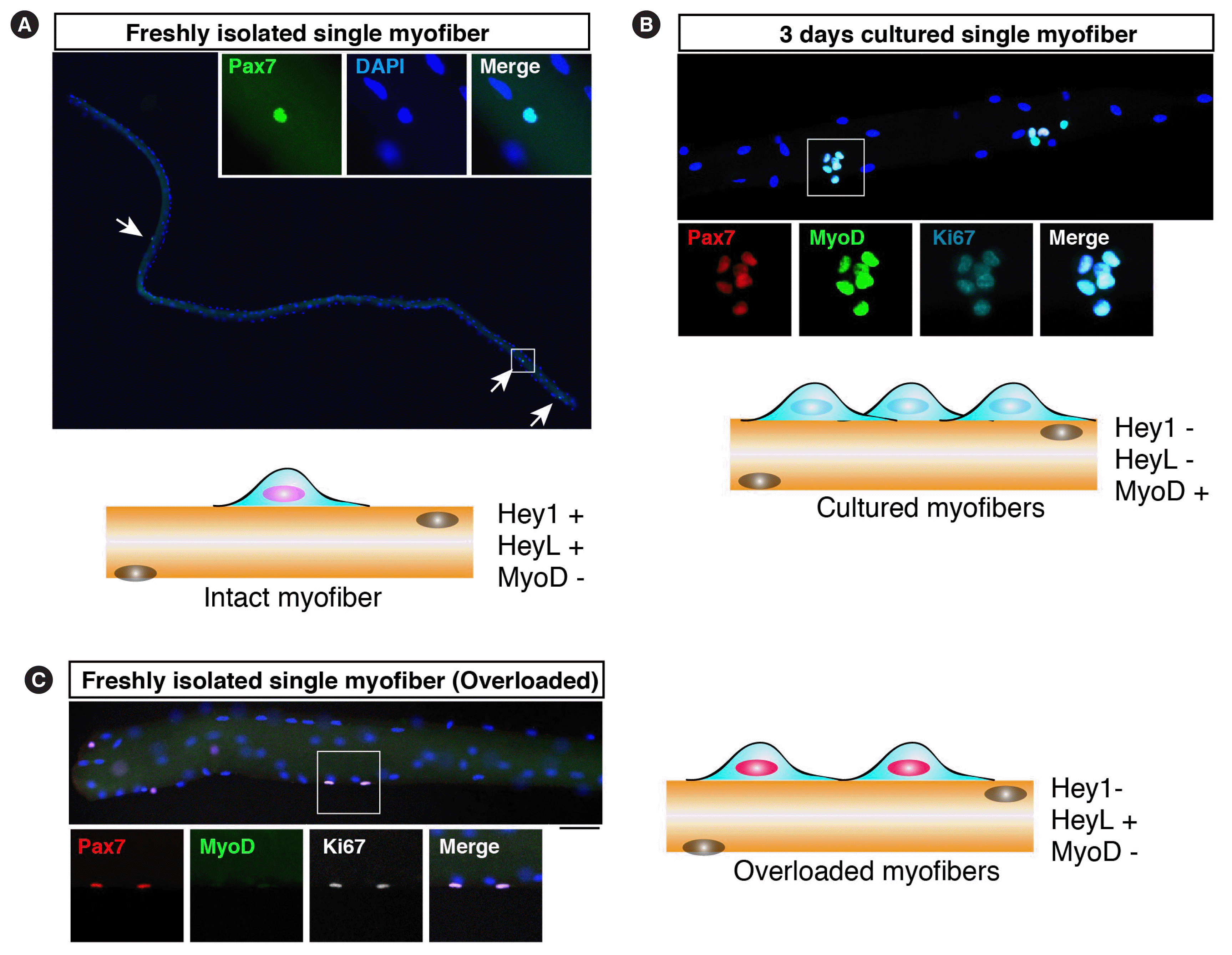
Table 1
List of Exercise or Exercise-Dependent Factor
Exercise/Exercise-dependent factor | Target | Function | Reference |
---|---|---|---|
Apelin | Myofiber, MuSCs | Anti-sarcopenia | [7] |
Apelin (maternal) | Fetal brown adipose tissue (BAT) | Enhance BAT development | [8] |
GDF15 | Adipose tissues | Lypolysis | [9] |
IL-6 | NK cells | Anti-tumor | [5] |
Kynurenine aminotransferases | Kynurenine in blood | Anti-depression | [4] |
SPARC | Colon cancer cells | Anti-tumor | [6] |
Succinate | Multiple types of cells in skeletal muscle | Muscle remodeling | [10] |