Abstract
Objective
Cerebral vessels, such as intracerebral perforating arterioles isolated from rat brain, have been widely used as an ex vivo model to study the cerebrovascular function associated with cerebrovascular disorders and the therapeutic effects of various pharmacological agents. These perforating arterioles, however, have demonstrated differences in the vascular architecture and reactivity compared with a larger leptomeningeal artery which has been commonly implicated in cerebrovascular disease. In this study, therefore, we developed the method for studying cerebrovascular function utilizing the olfactory artery isolated from the mouse brain.
Methods
The olfactory artery (OA) was isolated from the C57/BL6 wild-type mouse brain. After removing connective tissues, one side of the isolated vessel segment (approximately -500 µm in length) was cannulated and the opposite end of the vessel was completely sealed while being viewed with an inverted microscope. After verifying the absence of pressure leakage, we examined the vascular reactivity to various vasoactive agents under the fixed intravascular pressure (60 mm Hg).
Results
We found that the isolated mouse OAs were able to constrict in response to vasoconstrictors, including KCl, phenylephrine, endothelin-1, and prostaglandin PGH2. Moreover, this isolated vessel demonstrated vasodilation in a dose-dependent manner when vasodilatory agents, acetylcholine and bradykinin, were applied.
Conclusion
Our findings suggest that the isolated olfactory artery would provide as a useful ex vivo model to study the molecular and cellular mechanisms of vascular function underlying cerebrovascular disorders and the direct effects of such disease-modifying pathways on cerebrovascular function utilizing pharmacological agents and genetically modified mouse models.
Tissue-organ bath systems have been widely used in ex vivo studies for cerebrovascular function by measuring the forces in strips or rings of isolated vessel segments9,19,20,23,25), whereas the transcranial window methods have been developed to study the vessel function in vivo in live experimental rodents18), in particular, in leptomeningeal arteries and arterioles (only to a depth of 250 µm from the cortical surface)17,24). The ex vivo studies would have several advantages over the in vivo cranial window methods in the following respects : 1) this method allows dose-escalating and/or antagonism studies, 2) biochemical degradation and/or metabolism of applied agents is negligible, 3) the effects of nonvascular cells (i.e., glial and neuronal cells) can be removed, and 4) studies can be performed under physiologic as well as pathophysiological conditions. Larger pial arteries, where blood pressure and blow flow rate are tightly regulated, have been utilized in ex vivo studies largely because of the easiness of their preparation23,25). In addition, Dacey and Duling5) first demonstrated that small arterioles (i.e., perforating arterioles) served as an ex vivo experimental system though there were more technical difficulty as compared with the large vessel preparation.
The "vessel conduit system" utilizing intracerebral perforating arterioles isolated from rat brain has provided as a gold standard ex vivo model for many years4,5,7,9,12,13). Though this method with an organ bath setting is well suited for microvessel experiments, especially in terms of minimal mechanical injury during preparation5,9), utilization of larger vessels (i.e., leptomeningeal arteries form mouse brain) has not been addressed. For example, anatomically, intracerebral large subarachnoid arteries [as middle cerebral artery (MCA)] of rodents show a short interbranch distance relative to vessel diameter, which makes it difficult to find a long (0.5-1 mm), branch-free segment feasible for cannulation. In this study, we developed the method for isolation of the olfactory artery from the mouse brain and characterization of vascular reactivity.
All procedures were approved by the Washington University Advisory Committee for Animal Resources. Male C57/BL6 mice (10 to 14 weeks of age) were purchased from the Jackson Laboratory (Bar Harber, ME, USA) and anesthetized with sodium pentobarbital (30 mg/kg, i.p.). We isolated the olfactory artery per published protocol5,7,9). Briefly, the brain was rapidly removed cautiously without damaging vessels within the Circle of Willis and basal large arteries. The removed brain was faced to ventral surface up for the olfactory artery (OA)2) dissection in cooled (4℃) chamber with 3-(N-morpholino) propanesulfonic acid (MOPS)-buffered saline (in mmol/L : 144 NaCl, 3.0 KCl, 2.5 CaCl2, 1.4 MgSO4, 2.0 pyruvate, 5.0 glucose, 0.02 ethylenediaminetetraacetic acid, 1.21 NaH2PO4, and 2.0 MOPS) containing 1% dialyzed bovine serum albumin. Of note is that the OA is also named internal ethmoidal artery (IEA) (Fig. 1)2,8,27). Under a surgical microscope, both OAs were separated from the parenchyma and in turn an unbranched OA vessel segment (approximately 500 µm or longer) was prepared for cannulation.
The isolated vessel was carefully transferred with a Wiretrol II 5-10 µL micropipette (Drummond Scientific Co., Broomall, PA, USA) from dissection chamber to a temperature controlled cannulation chamber (2.5 mL) which was mounted on a stage of an inverted microscope (Zeiss TV 200, Thornton, New York, USA). The holding and perfusion pipettes used for cannulation were fabricated by pulling and shaping glass tubes (Drummond Scientific Co.; 2.13 mm O.D., 1.63 mm I.D., and 1.19 mm O.D., 1.02 mm I.D., respectively; 15-cm length) using a microforge (Stoelting Co., Wood Dale, IL, USA). The collecting pipettes were also fabricated from the same glass as the holding pipettes (2.13 mm O.D., 1.63 mm I.D.). The final holding pipette diameter was 80-90 µm and perfusion pipette diameter was 45-50 µm. The vessel was cannulated at one end with the concentrically mounted perfusion (inner) and holding (outer) pipette system. The opposite end was completely sealed by the collecting pipette. All experiments were performed at a fixed intravascular pressure (60 mm Hg) applied through the perfusion pipette without intraluminal flow (Fig. 2). After successful cannulation, the bath temperature was raised from room temperature to 37.5℃. We discarded vessels if there was a pressure drop. We equilibrated the cannulated artery in the organ bath for 45 minutes. Before the experiment, vessel responsiveness was tested by applying 50 mM KCl. If the vessel showed good response, we further investigated vessel responses to other vasoactive agents after washing out KCl thoroughly. If the vessel showed a poor response to 50 mM KCl (less than 15% diameter change), we discarded the vessel.
The organ bath was continuously circulated with MOPS buffer using a peristaltic pump (model 203, Scientific Industries, Bohemia, NY, USA) at a rate of 0.5 mL/min. The cannulation pipettes are mounted in custom made plexiglas holders, which have side ports to allow intraluminal pressure application and measurement as well as suction to aspirate the vessel. The plexiglas holders were mounted on a microscope mounted V-track system9). The artery was observed with the aid of a video system (DageMTI Inc., Michigan City, IN, USA) and displayed on a monitor. For measurement of the vessel diameter, a computerized diameter tracking system (Diamtrak software, Tim Neild, Adelaide, Australia) was used. The vessel diameter and pressure data were recorded and digitally stored continuously.
We applied all drugs dissolved in MOPS at 37.5℃ sequentially from low to high concentrations. After the vessel responsiveness was confirmed with KCl, we added phenylephrine (PE; Sigma, St. Louis, MO, USA), followed by prostaglandin PGH2 (U-46619; Sigma) or endothelin-1 (ET-1; Sigma, St. Louis, MO, USA). After applying of KCl and PE, we waited until the vessel diameter returned to the initial baseline by washing out the former drug completely at least for 20 minutes. For the dilation activity study, we induced submaximal constriction with PE 5×10-6 M. If the vessel showed good response with PE 5×10-6 M (more than 8% diameter change), we applied acetylcholine (ACh; Sigma, St. Louis, MO, USA) and bradykinin (BK; Sigma, St. Louis, MO, USA). If the vessel showed poor response less than 8% diameter change with PE 5×10-6 M, we discarded the vessel because it could be difficult to detect vasodilatory effect clearly.
Only one vessel was used from each animal. Values were expressed as mean±standard error of mean where n indicated the number of vessels studied. One-way repeated-measure analysis of variance (ANOVA) was performed to test for differences between concentration-response of KCl relationships. Agonist comparisons for concentration-response curves were analyzed with a two-way ANOVA with repeated measures using the PROC Mixed General Linear Models associated with the Statistical Analysis Software (SAS 9.3, SAS Institute, Inc., Cary, NC, USA). The Log (EC50) values (concentration producing 50% of the maximal response) were calculated by nonlinear regression analysis of concentration-response curves of each agonist (Prism 5.0, Graph Pad Software Inc., San Diego, CA, USA). We used the equation to calculate the percent of constriction as [(Dinitial-Ddrug)/Dinitial]×100%, where Dinitial is the diameter just before applying vasoconstrictor and Ddrug is the diameter after vasoconstrictor. Percent dilation was calculated using the equation [(Ddrug-Dbase)/(Dinitial-Dbase)]×100%, where Ddrug is the diameter in drug for vasodilator and Dbase is the diameter in drug for submaximal constriction and Dinitial is the diameter just before applying vasoconstrictor (PE 5×10-6 M). We considered a two-sided P<0.05 statistically significant.
The baseline diameter of mouse OAs in this study was 120±3.5 µm (n=28) at 60 mm Hg. Application of a depolarizing agent of KCl showed a dose-dependent vasoconstriction (n=12) (Fig. 3). The maximal constriction with 150 mM KCl was 46.3±3.9%. We then examine the response of OAs to the strong vasoconstrictor (n=20). We found there were two populations of vessels : 1) the vessel segments (n=15) having constriction by ≥8% in response to 1×10-5 M PE (28.6±3.8%) (Fig. 4), or 2) the vessel segments (n=5) having constriction by <8% in response to 1×10-5 M PE (2.6±0.6%), though all vessel segments revealed substantial vasoconstriction to KCl. U-46619 induced a maximal constriction (49.4±6.3%, n=9) at a concentration of 1×10-6 M (Fig. 4). The ET-1, known as a potent vasoconstrictor, showed most significant and prolonged vasoconstriction in most of the OAs tested with the greatest potency and efficacy among the vasoconstrictors tested (43.7±4.7% at 1×10-8 M, n=8) (Fig. 4).
Next, we examined the vasodilatory responses of the isolated OAs under the condition that the vessels were sub-maximally pre-constricted in the presence of 5×10-6 M PE. We found that both ACh and BK (1×10-11-1×10-4 M) induced vasodilation in a dose-dependent fashion. The maximal vasodilation was observed when the vessels were treated with 1×10-4 M Ach (54.7±6.3%, n=8) or 1×10-6 M BK (35.7±9.0%, n=8) (Fig. 5).
In the present study, we recorded the vascular reactivity of isolated mouse olfactory artery ex vivo which constricted in response to various vasoconstrictors, including KCl, PE, ET-1, and a thromboxane agonist (prostaglandin PGH2; U-46619). Moreover, this isolated vessel demonstrated vasodilation in a dose-dependent manner when treated with vasodilatory agents including acetylcholine and bradykinin. Our findings suggest that the isolated olfactory artery would provide as a useful ex vivo model to study the molecular and cellular mechanisms of vascular function underlying cerebrovascular disorders and the direct effects of such disease-modifying pathways on cerebrovascular function utilizing pharmacological agents and genetically modified mouse models.
Leptomeningeal arteries have distinct features morphologically and functionally as compared with perforating arterioles and capillaries in the brain. For example, leptomeningeal arteries (also called subarachnoid and pial arteries) are surrounded by the cerebrospinal fluid and have multiple layers of vascular smooth muscle cells (VSMCs), while smaller branches of cerebral vessels like perforating arterioles is consist of a single layer of VSMCs6,10). An inner elastic lamina is seen in the major leptomeningeal arteries but not in the perforating arterioles10). In mouse model of cerebral aneurysm, we can see the typical anatomic feature of large leptomeningeal artery at OA16). Large pial arteries are innervated, but perforating arterioles are not6). In fact, smaller perforating arterioles are closely communicated with surrounding glial cells and neuronal parenchymal elements, which leads to distinct vascular reactivity to vascular stimuli. In one hand, large leptomeningeal arteries are more sensitive to sympathetic nerve stimuli26), whereas small arterioles are more reactive than that of large arteries to a certain condition such as an elevation of pCO2 or hypercapnia11,15). On the other hand, large cerebral arteries are more sensitive to changes in blood pressure while cerebral blood flow in these vessels remains unchanged via an autoregulatory mechanism15). Thus, because of their anatomical and functional differences between large leptomengeal arteries and small perforating arterioles, one cannot extrapolate the findings from the large arteries to those of the small arterioles, or vice versa.
Dacey and Duling5) initially developed an elegant ex vivo experimental system where perforating arterioles isolated from rat brain was utilized. Since genetically modified mouse models (designed to either deplete or overexpress target proteins) have been more widely used in the many setting of neurovascular disease, ex vivo experiments utilizing such genetically modified mice are desired. However, the ex vivo experimental technique, in particular, using large leptomeningeal arteries of mice has not been fully established largely due to the technical difficulty in isolation of cerebral vessels without physical stress and cannulation of these leptomeningeal arteries. Though one study reported that perforating intracerebral arterioles demonstrates spontaneous tone and responses to vasoactive reagents3), we observed that these perforating arterioles from C57Bl6 mouse (the same type of mouse that Coyne et al.3) used in the study) often had many small branches of arterioles; therefore, it was difficult to maintain the intraluminal pressure (60 mm Hg) consistent throughout the experiment. Similarly, we found a large vessel segment of the proximal middle cerebral artery had the same issue. We, therefore, sought to examine what vessel segment of large leptomeningeal arteries in C57Bl6 mouse (the most common source strain of genetically engineered mice) would be more feasible for ex vivo studies without such technical problems.
We tried to find out unbranched intracerebral large artery that was easy to handle with proper size during vessel isolation and cannulation. During preliminary anatomical dissection study, OAs, which was also named IEAs had fewer branches and was suitable size to manipulate2,8,27). Also OA was remarkably constant in its origin, size and course in rat2) and it was similar in mouse, we found the olfactory segments from mouse brain showed slightly smaller diameter than that of the vessel segments from the proximal MCA (40-90 µm)1,22) before pressure loading. After cannulation and incubation with an intraluminal pressure of 60 mm Hg, these vessels passively dilated up to 120±3.5 µm in diameter by 45 min post incubation, which is also smaller to MCA diameter of 142.5±7.9 µm14). In contrast, it was reported that isolated perforating arterioles showed spontaneous constriction under the same bathing medium as our experimental condition during this period of incubation after cannulation3,5), indicating functional difference between leptomeningeal and perforating vessels. This notion is further supported by previous studies demonstrating that pial arteries and perforating arterioles responded differently to reperfusion after transient ischemia17). We found the isolated olfactory artery was capable to dilate as well as constrict in response to vasoactive agents (Fig. 4). In fact, vascular reactivity of the olfactory artery to these vasoactive agents is to some extent comparable to that of the MCA of mouse brain1,21,22). For example, the potent vasoconstrictor ET-1 induced vasoconstriction by 43.7±4.7% at a concentration of 1×10-8 M in the olfactory artery (Fig. 4) while it constricted the MCA by 47.8±4.2% at 5×10-8 M1). Interestingly, we found though most of the selected olfactory arteries (15 of 20) displayed consistent vasoconstrictor response 1×10-5 M of PE, the rest of vessels showed little response to PE. This variable response could be possible that the distribution of α-adrenergic receptors varies between the isolated olfactory arteries.
The limitations of this study are small number of experimental vessels and we added chemical agents only extraluminally. Moreover, our laboratory processes require learning period to be accustomed due to meticulous manipulation of vessels and handling of fine glass tube. Despite these limitations, our study suggests that isolated mouse OA is a useful ex vivo model of large leptomeningeal artery.
In the present study, we were able to isolate the olfactory arteries from mouse brain without causing apparent traumatic damage and measure the vessel reactivity to vasoactive agents, suggesting that mouse olfactory arteries can be used to study the molecular and cellular mechanisms of cerebrovascular function.
Acknowledgements
Statistical consultation was supported by Department of Biostatistics of the Catholic Research Coordinating Center.
References
1. Bai N, Moien-Afshari F, Washio H, Min A, Laher I. Pharmacology of the mouse-isolated cerebral artery. Vascul Pharmacol. 2004; 41:97–106. PMID: 15380735.


2. Brown JO. The morphology of circulus arteriosus cerebri in rats. Anat Rec. 1966; 156:99–106. PMID: 5970448.


3. Coyne EF, Ngai AC, Meno JR, Winn HR. Methods for isolation and characterization of intracerebral arterioles in the C57/BL6 wild-type mouse. J Neurosci Methods. 2002; 120:145–153. PMID: 12385764.


4. Dacey RG Jr, Bassett JE. Cholinergic vasodilation of intracerebral arterioles in rats. Am J Physiol. 1987; 253(5 Pt 2):H1253–H1260. PMID: 3479909.


5. Dacey RG Jr, Duling BR. A study of rat intracerebral arterioles : methods, morphology, and reactivity. Am J Physiol. 1982; 243:H598–H606. PMID: 7124967.
6. Dahl E. Microscopic observations on cerebral arteries. In : Cervós-Navarro J, Betz E, Matakas F, Wiillenweber R, editors. The Cerebral Vessel Wall. New York: Raven Press;1976. p. 15–21.
7. Dietrich HH, Kajita Y, Dacey RG Jr. Local and conducted vasomotor responses in isolated rat cerebral arterioles. Am J Physiol. 1996; 271(3 Pt 2):H1109–H1116. PMID: 8853348.


8. Dorr A, Sled JG, Kabani N. Three-dimensional cerebral vasculature of the CBA mouse brain : a magnetic resonance imaging and micro computed tomography study. Neuroimage. 2007; 35:1409–1423. PMID: 17369055.


9. Duling BR, Gore RW, Dacey RG Jr, Damon DN. Methods for isolation, cannulation, and in vitro study of single microvessels. Am J Physiol. 1981; 241:H108–H116. PMID: 7195654.


10. Edvinsson L, Krause DN. The blood vessel wall. Endothelial and smooth muscle cells. In : Edvinsson L, Krause DN, editors. Cerebral Blood Flow and Metabolism. ed 2. Philadelphia: Lippincott Williams & Wilkins;2002. p. 30–42.
11. Harper AM, Deshmukh VD, Rowan JO, Jennett WB. The influence of sympathetic nervous activity on cerebral blood flow. Arch Neurol. 1972; 27:1–6. PMID: 4626103.


12. Horiuchi T, Dietrich HH, Hongo K, Dacey RG Jr. Mechanism of extracellular K+-induced local and conducted responses in cerebral penetrating arterioles. Stroke. 2002; 33:2692–2699. PMID: 12411663.


13. Horiuchi T, Dietrich HH, Tsugane S, Dacey RG Jr. Role of potassium channels in regulation of brain arteriolar tone : comparison of cerebrum versus brain stem. Stroke. 2001; 32:218–224. PMID: 11136940.


14. Kidoguchi K, Tamaki M, Mizobe T, Koyama J, Kondoh T, Kohmura E, et al. In vivo X-ray angiography in the mouse brain using synchrotron radiation. Stroke. 2006; 37:1856–1861. PMID: 16741182.


15. Kontos HA, Wei EP, Navari RM, Levasseur JE, Rosenblum WI, Patterson JL Jr. Responses of cerebral arteries and arterioles to acute hypotension and hypertension. Am J Physiol. 1978; 234:H371–H383. PMID: 645875.


16. Morimoto M, Miyamoto S, Mizoguchi A, Kume N, Kita T, Hashimoto N. Mouse model of cerebral aneurysm : experimental induction by renal hypertension and local hemodynamic changes. Stroke. 2002; 33:1911–1915. PMID: 12105374.
17. Morita M, Ohkawa M, Miyazaki S, Ishimaru T, Umetani K, Suzuki K. Simultaneous observation of superficial cortical and intracerebral microvessels in vivo during reperfusion after transient forebrain ischemia in rats using synchrotron radiation. Brain Res. 2007; 1158:116–122. PMID: 17540351.


18. Rosenblum WI. A review of vasomotor responses of arterioles on the surface of the mouse brain : the necessary prelude to studies using genetically manipulated mice. Microcirculation. 1998; 5:129–138. PMID: 9789254.


19. Sasaki T, Kassell NF, Torner JC, Maixner W, Turner DM. Pharmacological comparison of isolated monkey and dog cerebral arteries. Stroke. 1985; 16:482–489. PMID: 2860741.


20. Toda N. Influence of dopamine and noradrenaline on isolated cerebral arteries of the dog. Br J Pharmacol. 1976; 58:121–126. PMID: 974370.


21. Tong XK, Hamel E. Transforming growth factor-beta 1 impairs endothelin-1-mediated contraction of brain vessels by inducing mitogen-activated protein (MAP) kinase phosphatase-1 and inhibiting p38 MAP kinase. Mol Pharmacol. 2007; 72:1476–1483. PMID: 17848599.


22. Tong XK, Nicolakakis N, Kocharyan A, Hamel E. Vascular remodeling versus amyloid beta-induced oxidative stress in the cerebrovascular dysfunctions associated with Alzheimer's disease. J Neurosci. 2005; 25:11165–11174. PMID: 16319316.


23. Uchida E, Bohr DF, Hoobler SW. A method for studying isolated resistance vessels from rabbit mesentery and brain and their responses to drugs. Circ Res. 1967; 21:525–536. PMID: 4293658.


24. Villringer A, Haberl RL, Dirnagl U, Anneser F, Verst M, Einhäupl KM. Confocal laser microscopy to study microcirculation on the rat brain surface in vivo. Brain Res. 1989; 504:159–160. PMID: 2598012.


25. Vinall PE, Simeone FA. Cerebral autoregulation : an in vitro study. Stroke. 1981; 12:640–642. PMID: 7303050.
26. Wei EP, Raper AJ, Kontos HA, Patterson JL Jr. Determinants of response of pial arteries to norepinephrine and sympathetic nerve stimulation. Stroke. 1975; 6:654–658. PMID: 1198630.


27. Wiland C. Comparative study on structure and variation in basal arteries of the brain in laboratory mouse. Anat Anz. 1974; 135:455–464. PMID: 4417827.
Fig. 1
Anatomical structure of leptomeningeal arteries in mouse brain. OA : olfactory artery (also called internal ethmoidal artery), ICA : internal carotid artery, ACA : anterior cerebral artery, MCA : middle cerebral artery, PCA : posterior cerebral artery, BA : basilar artery.
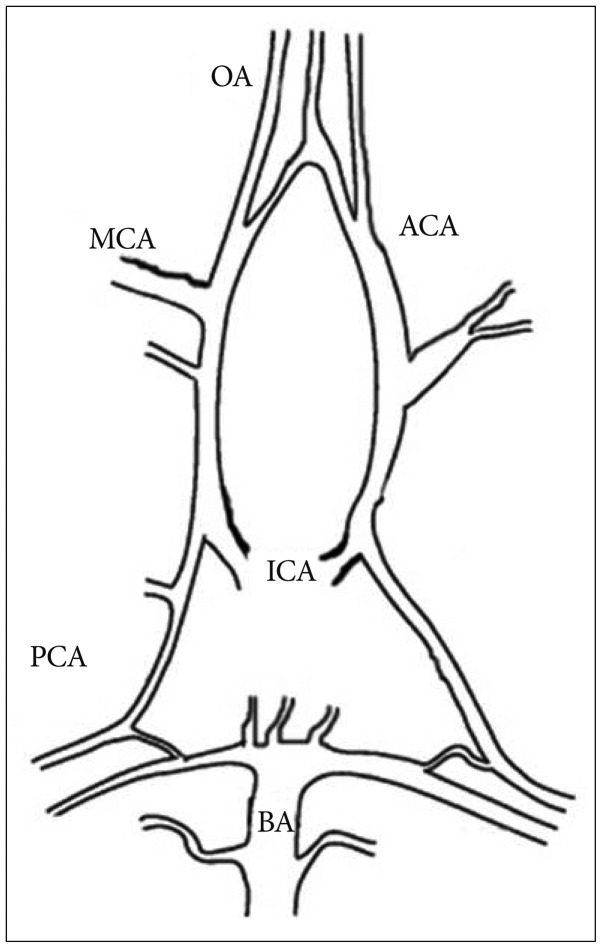
Fig. 2
Cannulated olfactory artery of mouse with fixed intraluminal pressure (60 mm Hg). Holding (arrowhead) and perfusion pipette (arrow) are located at right. Collecting pipette is shown at left.

Fig. 3
Vasoconstrictory response of isolated olfactory artery to the depolarizing agent of KCl (n=12).

Fig. 4
Vasoconstrictory response of isolated olfactory artery to phenylephrine (n=15), U-46619 (n=9), and endothelin-1 (n=8). The (-LogEC50) values of ET-1, U-46619, and PE were 8.95±inf, 7.66±0.29, and 5.43±0.17 M, respectively. The vasoconstriction values tended to increase over the concentration with all of the agonist (p≤0.0001). This difference was significant among the three agonist (p≤0.0001). ET-1 : endothelin-1, PE : phenylephrine.

Fig. 5
Vasodilatory response of isolated olfactory artery to acetylcholine (n=8) and bradykinin (n=8). The (-LogEC50) values of BK and ACh were 10.14±2.38 and 6.70±0.73 M, respectively. The vasoconstriction values tended to increase over the concentration with all of the agonist (p≤0.0001). This difference was significant between BK and ACh (p=0.0139). BK : bradykinin, ACh : acetylcholine.
