Abstract
Objective
There is a rich literature confirming the default mode network found compatible with task-induced deactivation regions in normal subjects, but few investigations of alterations of the motor deactivation in patients with intracranial lesions. Therefore, we hypothesized that an intracranial lesion results in abnormal changes in a task-induced deactivation region compared with default mode network, and these changes are associated with specific attributes of allocated regions.
Methods
Blood oxygenation level dependent (BOLD) functional magnetic resonance imaging (fMRI) during a motor task were obtained from 27 intracranial lesion patients (mean age, 57.3 years; range 15-78 years) who had various kinds of brain tumors. The BOLD fMRI data for each patient were evaluated to obtain activation or deactivation regions. The distinctive deactivation regions from intracranial lesion patients were evaluated by comparing to the literature reports.
Results
There were additive deactivated regions according to intracranial lesions: fusiform gyrus in cavernous hemangioma; lateral occipital gyrus in meningioma; crus cerebri in hemangiopericytoma; globus pallidus, lateral occipital gyrus, caudate nucleus, fusiform gyrus, lingual gyrus, claustrum, substantia nigra, subthalamic nucleus in GBM; fusiform gyrus in metastatic brain tumors.
Recent neuroimaging studies have described the default mode of brain function and spontaneous intrinsic low-frequency signal fluctuations during resting state. The default mode network is made up of brain regions that typically show higher signal levels when individuals are left to think to themselves undisturbed 14,15). It includes regions in the medial prefrontal cortex (ventromedial prefrontal cortex and anterior cingulated cortex), the medial parietal cortex (posterior cingulated cortex, precuneus, and retrosplenial cortex), and lateral temporo-parietal cortex (supramarginal gyrus, angular gyrus, and superior temporal sulcus). Its representative regions are summarized and listed in Table 1. These regions are compatible with task-induced deactivation regions in the study of functional imaging data based on signal changes compared to a baseline 13). The term "deactivation" was first used in the investigation of brain functions in 1990s with positron emission tomography (PET) studies. Scans of rest-state brain activity were often acquired across several studies for a control comparison, and researchers began to notice brain regions more active in the passive control conditions than the active target tasks4). The "default mode network" was described with a series of publications by Gusnard et al.7) and Raichle et al.15) in early 2000s. They distinguished between various forms of task-induced deactivation and separated deactivations defining the default network from other forms of deactivation. Furthermore, in the extension of this research, interest developed in connectivity and interaction between brain regions within a network or between networks. Recent methodological advances allowed both structural and functional connectivity to be studied in vivo2,9), and research regarding changes in deactivated regions with consequential modification of functional connectivity are at the point in investigation of human brain mapping.
Studies of the default mode using functional MRI (fMRI) technique have been carried out recently. The low-frequency fluctuation in resting state of fMRI signal intensity has been referred to the presence of spontaneous oscillations/fluctuations of the blood oxygenation level-dependent (BOLD) signal 10). Initial fMRI studies were concentrated on probing for signal changes in the low-frequency range for demonstrating a functional connectivity across hemisphere during rest 3,18). Subsequent studies have shown a functional connectivity for other sensory modalities 5) and language areas 8). Although there have not been enough studies dealing with motor task based deactivation, Allison et al.1), using motor task fMRI, first described interhemispheric control of motor action as involving deactivation to prevent interference from the opposite hemisphere. Marchand et al.11) described the detailed deactivated regions with respect to motor tasks and their functional connectivities based on normal human brain. Similar network analysis of default mode using fMRI has been reported up to the present day.
Nonetheless, there is still a lack of knowledge in the understanding of deactivations identities and roles during a task-induced BOLD fMRI study. Furthermore, only a few studies investigated alterations of the default mode network or deactivations in patients with intracranial lesions. Therefore, in this study, we investigated the alterations of the motor deactivation regions in patients with intracranial lesions compared with the normal status of brain. Therefore, we hypothesized that an intracranial lesion would cause changes in a task-induced deactivation region compared with the default mode network, and these changes are associated with changes in functional connectivity or plasticity.
Twenty-seven patients with intracranial lesions who underwent their pre-operative examination with fMRI only with motor task from July, 2007 to March, 2010 were retrospectively reviewed. Mean age was 57.3 years (range 15-78 years). Recruited intracranial disease entities were listed in Table 2. Language, memory, and sensory tasks were not analyzed for this study because there could be multiple influencing factors in those tasks according to intracranial lesions.
A 3.0-Tesla MRI system (Philips Achieva, Best, The Netherlands) was used. A patient was positioned supine, with the head in an eight channel head coil. The head was fixed by a sponge and the patient was not supposed to move his/her legs and head during the MRI scans. Transverse BOLD fMRI images were acquired with a gradient-echo echo-planar imaging sequence. The corresponding imaging parameters were: repetition time (TR)=3000 ms, echo time (TE)=35 ms, flip angle=90°, field of view (FOV)=230×230 mm, slice thickness=4 mm without interslice gap, number of slices=30, matrix size=64×64, and acquired voxel resolution=3.6×3.6×4.00 mm. In addition, anatomical images were acquired with a two-dimensional T2-weighted turbo spin echo sequence and a fluid attenuated inversion recovery (FLAIR) turbo spin echo sequence. The imaging parameters for the T2-weighted images were: TR=3000 ms, TE=250 ms, flip angle=90°, FOV=230×230 mm, slice thickness=3 mm, matrix size=256×256, acquired voxel resolution=0.9×0.9×3.00 mm, and transverse orientation. The imaging parameters for the FLAIR images were: TR=11000 ms, TE=140 ms, inversion time (TI)=2800 ms, flip angle=90°, FOV=230×230 mm, slice thickness=5 mm, matrix size=256× 256, acquired voxel resolution=0.9×0.9×5.00 mm, and transverse orientation. Finally, a three-dimensional (3D) T1-weighted (T1W) gradient echo sequence was run before and after injection of contrast agent with the following imaging parameters: TR=9.9 ms, TE=4.6 ms, flip angle=8°, FOV=240×240 mm, slice thickness=1 mm, acquired matrix size=240×240, voxel resolution=1.00×1.00×1.00 mm, and sagittal orientation.
Statistical Parametric Mapping 2 software (SPM2, Wellcome Department of Cognitive Neurology, London, UK) was used for post-processing of fMRI data. BOLD fMRI data were first realigned to correct head motion during the fMRI acquisition. Anatomical 3D T1W and fMRI images went co-registered. The 3D T1W images were spatially normalized into the Montreal Neurologic Institute (MNI) space and then fMRI images were also spatially normalized by using normalizing parameters of the 3D T1W image. The spatially normalized BOLD fMRI images were smoothed with the full-width half maximum of 8×8×8 mm to raise the power of discrimination among noises of MR signal changes classified by voxels produced by homodynamic response.
For the individual analysis of the smoothed BOLD fMRI data, activation and deactivation areas in the brain were obtained for each patient with the statistical threshold of p=0.001 with correcting multiple comparisons using the family wise error (FWE) method and with 10 voxels of cluster. We did not obtain group results because the locations of intracranial lesions were differed for each patient.
For each patient, we processed SPM maps of two single contrasts: 1) voxels having increased intensity during right-hand activity relative to rest; 2) voxels having increased intensity during left-hand activity relative to rest. "Deactivation" during right-hand activity in this study means that there was relatively less voxel intensity during right-hand activity than rest. Talairach transformation was performed for the complete set of functional data of each patient. To identify regions that are distinctively deactivated in motor task, we extract deactivated regions from representative default mode regions. Additionally, when deactivated regions are located near the brain lesion with a considerable surrounding edemaor mass effect which resulting in distortion of white matter tract, the deactivated regions are also excluded for avoiding confounding effect. Finally, we hired extracted deactivated regions for comparing with previously reported default mode network, and attempted to elucidate that these changes were correlated with specific attribute of allocated regions.
In response to non-dominant (left-hand) motor task, there were widespread deactivations of left postcentral gyrus, bilateral anterior cingulate gyri as well as right posterior cingulate, precuneus and middle temporal gyrus in most patients. Similar deactivations were observed in response to the dominant (right-hand) motor task, including areas in the bilateral posterior cingulate, left temporal lobe and cuneus as well as right pre-and postcentral gyri, and precuneus (Fig. 1).
However, there were 10 patients who exhibited additive motor task-induced deactivated regions (Fig. 2), and their detailed painformation is listed in Table 3. There were additive motor task-induced deactivated regions in one cavernous hemangioma (25%), 2 meningiomas (33.3%), 1 hemangiopericytoma (100%), 5 glioblastoma (45.5%), and 1 metastatic brain tumor (50%). There was no connection in lesion side and motor task side. However, there was a tendency of additive task-induced deactivated regions occurring at left motor task in glioblastoma patients to an appreciable extent.
When we summed and sorted regions, diverse regions appeared as additive task-induced deactivated regions for the motor task. Among them, globus pallidus, lateral occipital gyrus, fusiform gyrus, caudate nucleus were markedly deactivated and claustrum, precentral gyrus, and midbrain were also demonstrated for deactivated regions (Table 4). Caudate nucleus was distinctively deactivated in 2 glioblastoma patients. Two meningioma patients and 2 glioblastoma patients demonstrated additive deactivated regions in lateral occipital gyrus.
Fig. 3 shows MR images with a gadolinium enhancement and the representative deactivation map from the case 7 in the Table 3. MR images with a gadolinium enhancement revealed a large, well enhanced mass with central necrosis, which was sized 4.7×3.3×4.8 cm and located in right middle frontal gyrus (Fig. 3A). Task based fMRI scans demonstrated that the left hemisphere had localization similar to healthy individuals, while activity ipsilateral to the tumor was shifted postero-laterally suggesting displacement of cortical tissue involved in sensorimotor function. In response to left hand motor task, there were widespread deactivations in both cerebral hemisphere including post-central gyrus, precuneus, medial frontal cortex, and posterior cingulate gyrus, which were already known as default mode region. However, some regions other than default mode region demonstrated significantly reduced activations for the same task. They include middle occipital gyrus, caudate nucleus, fusiform gyrus, and lingual gyrus (Fig. 3B). In response to right hand motor task, there were also deactivated regions over the both cerebral hemisphere, but the regions were limited to default mode region.
Fig. 4 illustrate MR images with a gadolinium enhancement and the representative deactivation map from the case 4 in the Table 3. The MR imaging studies showed a well enhanced lobulating mass attached to the sagittal sinus in left frontoparietal lobe with a small cystic portion occupying mass in itself. In fMRI studies of left hand motor tasks, there were usual pattern of task-induced deactivations over the both hemispheres. In response to right hand motor task, there was unusual increased motor activity in right motor cortex, which didn't occur in left hand motor task, and motor activity in left motor cortex (ipsilateral to tumor) was shifted to anterior-laterally. Meanwhile, unusual reduced activation was appeared on the right crus cerebri in midbrain.
The aim of this study is was to describe motor deactivation, comparing normal brain with diseased brain. Motor task fMRI analysis delineated functional changes in brain networks in patients with intracranial disease. In line with a previous fMRI study of motor task based deactivation, we were able to identify motor task induced deactivations. They clarified ipsilateral (to the task) postcentral gyrus connectivity with the ipsilateral primary motor cortex for both hands, and found that motor deactivation was likely a motor-specific process with no relation to basal ganglia circuit deactivation. Consequently, motor task specific deactivations and their representative regions were included in our study and listed in Table 2.
We found several unique motor-task induced deactivated regions which do not occur to normal brains. Among the result of additive deactivated regions, the locations which appeared often were caudate nucleus, globus pallidus, lateral occipital gyrus, and fusiform gyrus.
Caudate nucleus was distinctively deactivated in 2 glioblastoma patients. Caudate nucleus is one of the functional basal ganglia loops which initiate the direct and indirect pathways of the basal ganglia. However, recent findings by Villablanca 17) suggested that it controls approach-attachment behavior and contributes to function sharing with the frontal cortex. Because the pathologic lesions (e.g., glioblastoma) were located in a superior portion of caudate nucleus, in this connection, this appears to be white matter tract alteration between basal ganglia loops and the frontal cortex.
Two meningioma patients and 2 glioblastoma patients demonstrated additive deactivated regions in lateral occipital gyrus. The occipital lobe is the visual processing center of the human brain containing most of the anatomical region of the visual cortex. The lateral occipital gyrus is at the front edge of occipital lobe component, which are separated by lateral occipital sulcus and it constitutes extrastriate cortex which is located next to the primary visual cortex. In terms of Brodmann areas, the extrastriate cortex comprises Brodmann area 18 and Brodmann area 19. The role of extrastriate cortex is the visual processing of static and moving images of the human body in more integrative and dynamic manner, being able to detect an incongruence of internal body or action representations and external visual signals 6). Martino et al. 12) described the anatomical connection between frontal lobe and occipital lobe using cadevaric research. They clarified the exact anatomical pathway of the inferior fronto-occipital fasciculus, and subdivided it into superficial and deep subcomponent. The connection between the frontal lobe with posterior portion of the inferior occipital gyrus and posterior portion of the fusiform gyrus consists of deep inferior frontal-occipital fasciculus. In this respect, our 4 patients with lateral occipital gyrus deactivation having all pathologic lesions around frontal lobe, the most likely influence was the inferior frontal-occipital fasciculus.
The fusiform gyrus is part of the temporal lobe in Brodmann area 37. It is located beneath the parahippocampal gyrus and above the occipitotemporal gyrus. The function of fusiform gyrus is still obscure and being researched by novel noninvasive means. However, recent reports regarding the role of fusiform gyrus offer some conclusive results as follows: 1) processing of color information, 2) face and body recognition, 3) word recognition, 4) number recognition, and 5) within-category identification. We found 1 patient with cavernous hemangioma and 1 glioblastoma patient showing deactivation upon fusiform gyrus. The cause for deactivation could be explained as the inferior frontal-occipital fasciculus connection for the glioblastoma patient. However, in case of the cavernous hemangioma, because the lesion was located in post-central gyrus of parietal lobe, the exact reason for deactivation is unknown.
In summary, although these phenomena appeared to be relatively specific changes in functional connectivity and brain network function, the cumulative effect of widespread white matter tract alteration across many tracts is likely to be a crucial factor affecting brain network function, rather than focal change in a particular location. With this, mood and cognitive changes, in which functional locations are not in proximity with the intracranial lesion, could be interpreted as extension of this process.
As for the changes in deactivated region, Sharp et al.16) proposed that there are two interrelated processes. One is a compensatory increase in functional connectivity within the default mode network and another is a variable degree of structural disconnection that modulates functional connectivity within the default mode network. In this way, the interaction between these factors means that patients with intracranial lesions would be unable to maintain increased default mode network functional connectivity, either because of alteration in the internal connections of the default mode network, or to its connections with other networks. Therefore, to maintain normal default mode network or other functional network connectivity, a new region that constitute dysfunctional region is required, apparently generated by neuro-plasticity.
This study has some limitations. First, the study subjects had diverse intracranial lesions in types, locations, and lesion size. Because every kind of intracranial lesion has its own disease characteristics and behavior pattern, the uniformity of altered deactivation is very limited and could give erroneous conclusions. Further studies with larger sample size and well-sorted disease categorization will be needed to resolve this problem. Second, our study relies just on motor functional connectivity, not including language, memory, and sensory. Further studies should focus on each of functional connectivity and altered deactivation along its functional tasks. Third, our study used a cross sectional design. We studied patients with intracranial lesions before the surgery, so that altered deactivation could be found in the middle of generation. A longitudinal investigation would help with a better understanding of the default mode network. Finally, any retrospective study with data collection from medical records carries the risk of selection bias. For the investigation of default mode network for diseased brain with fMRI, we specifically limited our analysis within motor tasks because there could be many confounding factors.
The findings of the present study show, for the first time, that intracranial lesions altered motor-task deactivated regions. The additive deactivated regions in intracranial lesion patients provide preliminary evidence that a variable degree of structural changes modulate functional connectivity within the default mode network. It will be important to compare structurally changed brain with neuropsychiatric disorder which had been investigated extensively to enhance our understanding in human brain functional connectivity.
Acknowledgements
This work was supported by a grant from Kyung Hee University in 2009 (KHU-20090585).
References
1. Allison JD, Meador KJ, Loring DW, Figueroa RE, Wright JC. Functional MRI cerebral activation and deactivation during finger movement. Neurology. 2000; 54:135–142. PMID: 10636139.


2. Beckmann CF, DeLuca M, Devlin JT, Smith SM. Investigations into resting-state connectivity using independent component analysis. Philos Trans R Soc Lond B Biol Sci. 2005; 360:1001–1013. PMID: 16087444.


3. Biswal B, Yetkin FZ, Haughton VM, Hyde JS. Functional connectivity in the motor cortex of resting human brain using echo-planar MRI. Magn Reson Med. 1995; 34:537–541. PMID: 8524021.


4. Buckner RL, Andrews-Hanna JR, Schacter DL. The brain's default network: anatomy, function, and relevance to disease. Ann N Y Acad Sci. 2008; 1124:1–38. PMID: 18400922.
5. Cordes D, Haughton VM, Arfanakis K, Wendt GJ, Turski PA, Moritz CH, et al. Mapping functionally related regions of brain with functional connectivity MR imaging. AJNR Am J Neuroradiol. 2000; 21:1636–1644. PMID: 11039342.
6. David N, Cohen MX, Newen A, Bewernick BH, Shah NJ, Fink GR, et al. The extrastriate cortex distinguishes between the consequences of one's own and others' behavior. Neuroimage. 2007; 36:1004–1014. PMID: 17478105.


7. Gusnard DA, Raichle ME, Raichle ME. Searching for a baseline: functional imaging and the resting human brain. Nat Rev Neurosci. 2001; 2:685–694. PMID: 11584306.


8. Hampson M, Peterson BS, Skudlarski P, Gatenby JC, Gore JC. Detection of functional connectivity using temporal correlations in MR images. Hum Brain Mapp. 2002; 15:247–262. PMID: 11835612.


9. Jones DK. Studying connections in the living human brain with diffusion MRI. Cortex. 2008; 44:936–952. PMID: 18635164.


10. Krüger G, Glover GH. Physiological noise in oxygenation-sensitive magnetic resonance imaging. Magn Reson Med. 2001; 46:631–637. PMID: 11590638.


11. Marchand WR, Lee JN, Thatcher JW, Thatcher GW, Jensen C, Starr J. Motor deactivation in the human cortex and basal ganglia. Neuroimage. 2007; 38:538–548. PMID: 17888686.


12. Martino J, Brogna C, Robles SG, Vergani F, Duffau H. Anatomic dissection of the inferior fronto-occipital fasciculus revisited in the lights of brain stimulation data. Cortex. 2010; 46:691–699. PMID: 19775684.


13. Mayer JS, Roebroeck A, Maurer K, Linden DE. Specialization in the default mode: Task-induced brain deactivations dissociate between visual working memory and attention. Hum Brain Mapp. 2010; 31:126–139. PMID: 19639552.


14. Mazoyer B, Zago L, Mellet E, Bricogne S, Etard O, Houdé O, et al. Cortical networks for working memory and executive functions sustain the conscious resting state in man. Brain Res Bull. 2001; 54:287–298. PMID: 11287133.


15. Raichle ME, MacLeod AM, Snyder AZ, Powers WJ, Gusnard DA, Shulman GL. A default mode of brain function. Proc Natl Acad Sci U S A. 2001; 98:676–682. PMID: 11209064.


16. Sharp DJ, Beckmann CF, Greenwood R, Kinnunen KM, Bonnelle V, De Boissezon X, et al. Default mode network functional and structural connectivity after traumatic brain injury. Brain. 2011; 134(Pt 8):2233–2247. PMID: 21841202.
17. Villablanca JR. Why do we have a caudate nucleus? Acta Neurobiol Exp (Wars). 2010; 70:95–105. PMID: 20407491.
18. Xiong J, Parsons LM, Gao JH, Fox PT. Interregional connectivity to primary motor cortex revealed using MRI resting state images. Hum Brain Mapp. 1999; 8:151–156. PMID: 10524607.


Fig. 1
Map of motor task-induced activation differences in patient with cavernous hemangioma. Voxels showing significant brain activation during left hand motor task shown in yellow. Voxels showing significantly reduced activation for the same task shown in light-blue. Deactivations were observed in prefrontal cortex, posterior cingulated, precuneus, and postcentral gyrus.
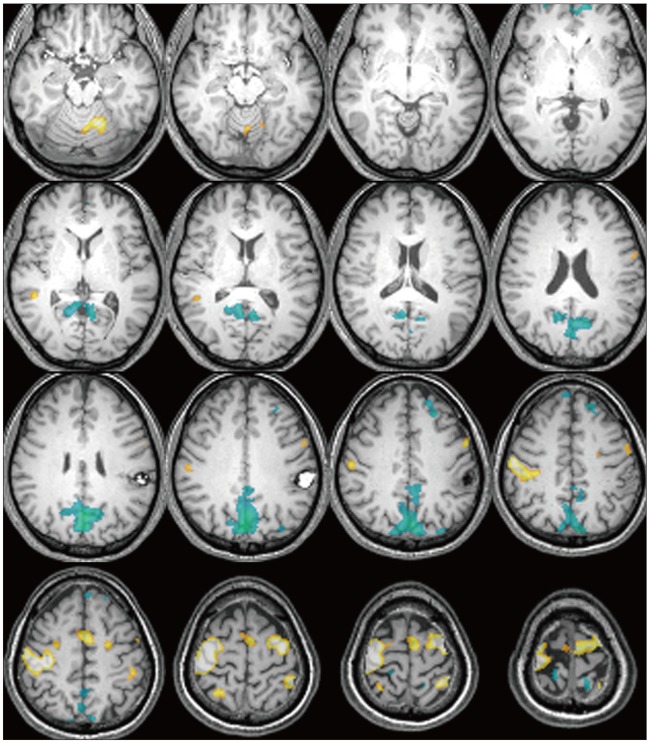
Fig. 2
Functional magnetic resonance imagings (fMRI) of the patients with intracranial lesions during motor task revealed additive task-induced deactivated regions. The case number is in the left lower corner of each patient's image. Distinctive deactivated regions are marked with arrowhead.

Fig. 3
MRI and fMRI in a 56-year-old woman with glioblastoma (case 7). A: T1-weighted MRI with gadolinium demonstrating a large, well enhanced mass with central necrosis in the right middle frontal gyrus, which is compatible with gliobalstoma. B: Axial fMRI series during left hand motor task showing activation (orange) and deactivation (light-blue) regions. Arrowhead denote the distinctive deactivated regions.
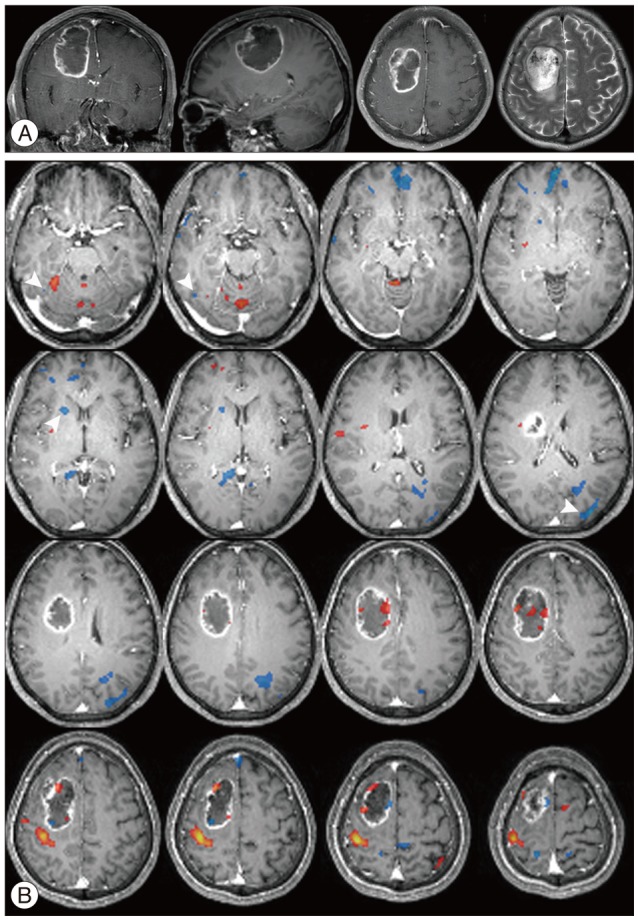
Fig. 4
MR imaging studies in a 53-year-old man with hemangiopericytoma (case 4). A: T1-weighted MRI with gadolinium demonstrating well enhanced lobulating mass adhering to sagittal sinus in left frontoparietal lobe. B: Axial fMRI series during right hand motor task showing activation (orange) and deactivation (light-blue) regions. Note that distinctive deactivated region in the right crus cerebri in midbrain (arrowhead).
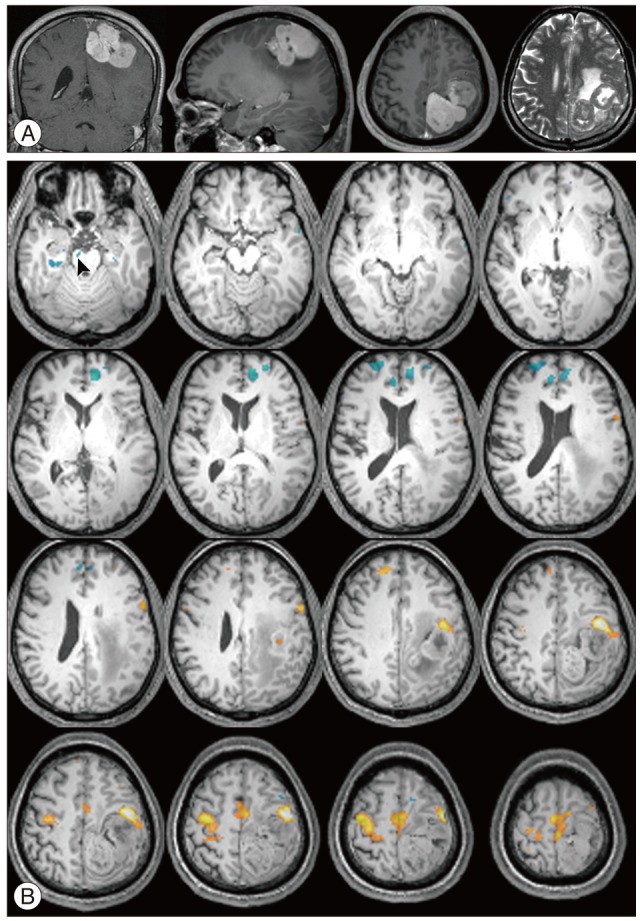