Abstract
Diabetes mellitus is a metabolic disorder resulting from an inadequate mass of insulin-producing pancreatic beta cells. The replacement or restoration of damaged beta cells would be considered the optimal therapeutic options. Islet transplantation seems to be a promising approach for replacement therapy; however, the main obstacle is the shortage of organ donors. As mature beta cells have been shown to be difficult to expand in vitro, regeneration of beta cells from embryonic or adult stem cells or pancreatic progenitor cells is an attractive method to restore the islet cell mass. So far, multiple studies using various strategies have shown direct differentiation of stem and progenitor cells toward insulin-producing cells. The important issue to be solved is how to differentiate these cells into mature functional insulin-producing cells. Further research is required to understand how endogenous beta cells differentiate and to develop methods to regenerate enough functional beta cells for clinically applicable therapies for diabetes.
Diabetes mellitus is one of the major chronic metabolic diseases, affecting at least 200 million people worldwide (1). According to the statistics of the Korean Diabetes Association, the prevalence of diabetes in Korea was 1% in 1970 and is estimated to be 14.4% in 2030 (http://www.diabetes.or.kr). Diabetes is characterized by uncontrolled hyperglycemia, which causes serious clinical problems including blindness, kidney failure, stroke, heart disease, and vascular disease.
Diabetes results from absolute or relative insulin deficiency. Type 1 diabetes is caused by autoimmune-mediated pancreatic beta cell destruction, and type 2 diabetes is caused by both insulin resistance in peripheral tissues and impaired beta cell function. Therefore, restoration of insulin-producing beta cells would be a logical strategy for the cure of both forms of diabetes. Injection of insulin is a way to treat diabetes, but it can have side effects such as hypoglycemic episodes. Islet transplantation is considered to be an effective method for the control of type 1 diabetes; however, it is hampered by limited donor organ availability.
Thus, recent studies have focused on generation of insulin-producing cells from renewable and limitless stem cells, which are able to differentiate into specialized cell types. There are two types of stem cells: embryonic stem cells and adult stem cells. Mesenchymal stem cells in adult tissues such as liver, adipose tissue, bone marrow, and intestine, and the umbilical cord blood stem cells have been reported to have the potential to differentiate into insulin-producing cells. In this review, we will discuss the differentiation of insulin-producing cells from adult stem/progenitor cells in pancreatic and non-pancreatic tissues.
The pancreas is composed of endocrine and exocrine tissues. The exocrine tissue occupies more than 80% of the pancreas and consists of acinar and ductal cells. The endocrine pancreas occupies less than 5% of the pancreatic tissue mass and is composed of cell clusters called the islets of Langerhans. The islets of Langerhans contain insulin-producing beta cells (about 80% of the cells in the islets), glucagon-producing alpha cells, somatostatin-producing delta cells, pancreatic polypeptide-producing cells, and ghrelin-producing epsilon cells.
During embryogenesis, gastrulation results in three principal germ layers: ectoderm, mesoderm, and endoderm. The endoderm germ layer forms the foregut, which then gives rise to the thyroid, lungs, liver, stomach, and pancreas. Following pancreas specification and budding, the cells proliferate and differentiate into endocrine and exocrine cells, and eventually form a fully developed pancreas (reviewed in 2, 3). Both extrinsic signals and intrinsic signals are involved in the programming of pancreatic endocrine cell differentiation. Extrinsic signals include intercellular signalings, and intrinsic signals include various transcription factors. Fibroblast growth factor (FGF), retinoic acid, and hedgehog signaling pathways are required for establishing the pancreatic organ within the developing gut tube, and transcription factors such as SRY (sex determining region Y)-box (Sox)17, homeobox gene HB9 (Hlxb9), hepatocyte nuclear factor (HNF)-6, HNF-3beta (also known as forkhead box A2, Foxa2), and pancreatic and duodenal homeobox 1 (Pdx-1) are required for proper pancreatic development (2, 3). Sox17 is the earliest specific marker of definitive endoderm and is required for pancreas formation. Hlxb9 is a critical factor for pancreatic endoderm development. Pancreas-specific transcription factor-1a (Ptf-1a) is known to be responsible for ventral pancreas specification.
Expansion and differentiation of pancreatic progenitor cells appear to be regulated by Notch signaling (4). FGF-10 is the mesenchymal signal and is important for expansion of Pdx-1-positive pancreatic progenitor cells (5). Notch signaling regulates the expression of neurogenin-3 (Ngn-3), which is a key regulator of pancreatic endocrine development and is expressed exclusively in endocrine precursor cells (6). Many transcription factors such as Pdx-1, ISL LIM homeobox 1 (Isl-1), NK2 homeobox 2 (Nkx2.2), NK6 homeobox 1 (Nkx6.1), neurogenic differentiation factor (NeuroD), Hlxb9, paired box gene (Pax)4, and Pax6 have been identified as islet differentiation factors. Much progress has been made on pancreas developmental biology including transcriptional regulation of pancreatic endocrine specification, growth, and lineage allocation, which contributes to our understanding of how to develop a method for efficient differentiation of pancreatic beta cells.
Bone marrow-derived stem cells are attractive source for differentiation protocols because these cells can be obtained easily from the patients. Many reports suggested that bone marrow cells have capacity to differentiate into multiple lineages, such as the hematopoietic lineage, endothelial cells, and mesenchymal cells. There have been controversial reports regarding the differentiation of pancreatic beta cells from bone marrow-derived stem cells. Ianus et al. reported that transplanted bone marrow cells differentiated into functional pancreatic beta cells in vivo without cell fusion (7). In this experiment, male mouse bone marrow cells that expressed green fluorescent protein (EGFP) when the insulin gene transcription was induced were transplanted into lethally irradiated female mouse recipients. The investigators detected EGFP+/insulin-positive cells in the pancreas of recipient mice at 4∼6 weeks after transplantation. However, other investigators could not reproduce these results (8, 9), suggesting that there is no evidence of transdifferentiation of bone marrow cells into pancreatic beta cells. Instead, it was suggested that the transplanted bone marrow cells secrete unknown factors that support the regeneration of pancreatic beta cells in the recipients. However, as all these studies used unfractionated heterogeneous bone marrow cells, it is not known which specific cell type contributed to the differentiation into insulin-producing cells or to regeneration of endogenous pancreatic beta cells.
As bone marrow cells contain two distinct stem cell population, hematopoietic stem cells (HSCs) and mesenchymal stem cells (MSCs), Tang et al. used single-cell-derived, bone marrow-derived MSCs from mice for in vitro differentiation and found that the differentiated cells expressed pancreas-specific marker genes (10). When these differentiated cells were transplanted into streptozotocin (STZ)-induced diabetic mice, hyperglycemia was reversed within 1 week. Others also showed that bone marrow-derived MSCs could trans-differentiate into insulin-producing cells by defined culture conditions (11). In contrast, another study demonstrated that transplantation of human bone marrow-derived MSCs into STZ-induced diabetic non-obese diabetic (NOD)/severe combined immune-deficient (scid) mice increased the number of endogeneous mouse beta cells and concomitantly increased mouse insulin, suggesting a supportive role of MSCs for regeneration of endogeneous beta cells (12).
Some papers reported that bone marrow-derived MSCs could be differentiated into insulin-producing cells by genetic manipulation. Overexpression of Pdx-1 in human bone marrow-derived MSCs resulted in differentiation into insulin-producing cells (13). Human bone marrow-derived MSCs transfected with three genes, Pdx-1, NeuroD, and Ngn-3, could be differentiated into insulin-expressing cells in vitro, but lacked glucose-responsive insulin expression. However, transplantation of these differentiated cells reduced blood glucose levels in diabetic mice. In a recent report, Luo et al. sorted several bone marrow subpopulations from GFP-positive mice, treated them with cytokines such as IL-3, IL-6, IL-11 and steel factors in vitro, and transplanted them into irradiated GFP-negative mice. They found that cytokine-treated bone marrow Sca+ subpopulations migrated to the pancreatic islets and differentiated into insulin-producing cells in vivo (14). However, the detailed mechanisms by which this process occurs remains to be determined. Although there are controversial reports about the transdifferentiation capacity of bone marrow cells, bone marrow-derived stem cells are considered important source for cell therapy for type 1 diabetes.
Adipose tissue-derived stem cells (ADSCs) are located within the stromal vascular fraction of adipose tissue. ADSCs can be isolated in high numbers from human adipose tissue at low risk to the patient. The cells have the potential to differentiate into adipogenic, osteogenic, chondrogenic, and myogenic cells when they are cultured in specific medium conditions. However, only a few studies have reported on the capability of ADSCs as a renewable source of insulin-producing cells (Fig. 1). It was reported that human ADSCs can differentiate into insulin-producing cells in vitro under specific medium conditions and that these cells express pancreatic developmental genes including Isl-1, Ipf-1, and Ngn-3 as well as the islet hormone genes, glucagon and somatostatin (15), although studies on the function of these differentiated cells are lacking. A recent study differentiated human adipose-derived MSCs from donors into insulin-producing cells by culturing them for 3 days using the same protocol and then transfused these differentiated cells together with cultured bone marrow-derived hematopoietic stem cells into diabetic patients by intraportal infusion. The patients showed a 30 % to 50 % decreased in their insulin requirement with a 4-to 26-fold increase in serum C-peptide levels, suggesting that transplantation of differentiated ADSCs may be an effective therapeutic method for treatment of diabetes (16).
Using a 3-step differentiation protocol, Chandra et al. reported that murine ADSCs differentiated into functional islet-like cell aggregates (ICAs) (17). They utilized a 10-day protocol in which mesodermic ADSCs were converted to definitive endoderm, then to pancreatic endoderm, and then to pancreatic hormone-expressing ICAs. The differentiated ICAs expressed endocrine genes and secreted C-peptide after glucose challenge. Transplantation of the mature ICAs intraperitoneally to STZ-induced diabetic mice restored normoglycemia within 2 weeks. An interesting study reported the successful differentiation of functional insulin-producing cells from stem cells of the human eyelid adipose tissue using a two-step culture condition (18). Similar to other stem cells, Pdx-1-transduced ADSCs derived from human or murine tissue could differentiate into insulin-expressing cells under specific culture conditions. (19). Thus, ADSCs have potential as a useful source for cell replacement therapy in diabetes.
Umbilical cord blood (UCB)-derived MSCs may be an ideal source of stem cells that can be obtained without pain and risk of viral contamination to the donor. UCB-derived MSCs have multi-lineage differentiation capacity under specific manipulation of the culture conditions, and several studies have shown that UCB stem cells can be differentiated into insulin-producing cells. UCB-derived embryonic stem cell-like cells which express stage-specific antigen 4 (SSEA4), octamer 4 (Oct4) could differentiate into insulin-producing islet-like cells. These islet-like cells expressed insulin and C-peptide protein (20). In addition, Denner et al. reported that human UCB-derived stem cells could become insulin-producing cells in vitro (21). Another study showed that the insulin-producing cells differentiated from UCB-derived MSCs with extracellular matrix gel secreted insulin but are not responsive to glucose (22). Furthermore, Yoshida et al. proved the regenerative property and the differentiation of UCB-derived cells to insulin-producing cells (23). When they transplanted UCB stem cells into immune deficient NOD/scid mice by intravenous injection, they could detect human UCB-derived insulin-positive cells in the pancreas of the recipient. These results suggest that UCB stem cells can differentiate into insulin-producing cells both in vitro and in vivo.
Adult stem/progenitor cells from liver tissue are good source for making insulin-producing cells, as liver and pancreas share common bipotential precursor cells within the embryonic endoderm. Many studies have demonstrated that adult hepatocytes, human fetal liver cells, and hepatic stem cells can be differentiated into insulin-producing cells by forced expression of beta cell transcription factors and/or manipulating the external microenvironment.
Jin et al. recently reported that when immortalized liver epithelial progenitor cells, derived from regenerative liver, were stably transduced with Pdx-1, followed by treatment with various growth factors they differentiated into insulin-expressing cells. These cells secreted insulin in response to glucose, and transplantation of these cells ameliorated diabetes in STZ-induced diabetic scid mice (24). Ectopic expression of Pdx-1 in the liver of mice induced insulin expression and ameliorated STZ-induced diabetes (25). Expression of Pdx1-VP16, a constitutive active form of Pdx-1, in the liver together with NeuroD or Ngn-3, more efficiently induced the differentiation of hepatocytes into insulin-expressing cells (26).
Rat hepatic oval stem cells, which can differentiate into hepatocytes and bile duct epithelium, differentiate into pancreatic endocrine cells when cultured in a high glucose environment, secrete insulin in response to glucose, and have the ability to reverse hyperglycemia in STZ-induced diabetic NOD/scid mice (27). Human fetal liver progenitor cells expressing Pdx-1 can convert to insulin-expressing cells (28).
Numerous studies suggest the existence of stem/progenitor cells in adult pancreatic ducts. Islet cells are observed in close proximity to ducts in type 1 diabetic patients and partially pancreatectomized rodents (29, 30). It was found that islet-like aggregates are generated from mouse pancreatic ducts and ductal tissue-enriched human pancreatic islets, and these aggregates release insulin after glucose stimulation and express islet proteins (31, 32). Ectopic expression of Ngn-3 in pancreatic ductal cells converted them into insulin-producing cells, and treatment of human islets containing both ductal and acinar cells with a combination of epidermal growth factor and gastrin induced neogenesis of islet beta cells from the ducts and increased the functional beta cell mass (33).
Hao et al. demonstrated that purified human non-endocrine pancreatic epithelial cells differentiated into endocrine cells when co-transplanted with human fetal pancreatic tissue under the kidney capsule of immunodeficient mice. Fetal cells seemed to provide factors that supported the survival and differentiation of the epithelial cells (34). A recent study demonstrated that progenitor cells expressing Ngn-3, which is hardly expressed in normal postnatal pancreatic tissues, exist in the ducts of the adult mouse pancreas. The study also found that isolated Ngn3-positive cells from the injured adult mouse pancreas differentiated into glucose-responsive insulin-producing beta cells (35). Another study also demonstrated that clonally identified cells from adult pancreatic islets and ductal populations have the ability to differentiate into cells with a beta cell function (36).
Mesenchymal cells derived from human islet preparations have been proposed as islet precursor cells. Considerable evidence suggests that beta cells in the pancreatic islets can be dedifferentiated, expanded, and redifferentiated into beta cells by inducing the epithelial-mesenchymal transition process (37). However, recent studies from lineage analysis suggest that the putative mesenchymal islet precursors are not derived from beta cells (38, 39).
In addition, amylase- and elastase-positive acinar cells can transdifferentiate into insulin-producing cells (40). An alpha cell line transfected with Pdx-1 could express insulin when treated with betacellulin. These results suggest that stem/progenitor cells exist within the pancreas, and these cells might be the source of new islets. However, the identification of specific markers is definitely needed for isolation of these cell populations.
Adult stem cells are the most important source for cell therapy of various disease models, as they are free from ethical problems and provide an unlimited resource. Many studies reported that insulin-producing cells can be generated from stem/progenitor cells present in bone marrow, adipose tissue, liver, intestine, spleen, salivary glands, neuronal tissues, and umbilical cord blood. Despite the success of the differentiation protocols as described in this review, none of the protocols are yet reproducible for the production of fully functional mature beta cells. Much research for the development of more sophisticated differentiation protocols is still required to apply these strategies clinically, but the success of the generation of glucose-responsive insulin-producing cells give hope for a treatment of diabetes by stem cell- based cell therapy in the future.
ACKNOWLEDGMENTS
This work was supported by a grant of the Korea Healthcare technology R&D project, Ministry for Health, Welfare & Family Affairs, Republic of Korea (A062260). We thank Ann Kyle for editorial assistance.
References
1. Wild S, Roglic G, Green A, Sicree R, King H. Global prevalence of diabetes: estimates for the year 2000 and projections for 2030. Diabetes Care. 2004. 27:1047–1053.


2. Jørgensen MC, Ahnfelt-Rønne J, Hald J, Madsen OD, Serup P, Hecksher-Sørensen J. An illustrated review of early pancreas development in the mouse. Endocr Rev. 2007. 28:685–705.


3. Murtaugh LC. Pancreas and beta-cell development: from the actual to the possible. Development. 2007. 134:427–438.


4. Hald J, Hjorth JP, German MS, Madsen OD, Serup P, Jensen J. Activated Notch1 prevents differentiation of pancreatic acinar cells and attenuate endocrine development. Dev Biol. 2003. 260:426–437.


5. Bhushan A, Itoh N, Kato S, Thiery JP, Czernichow P, Bellusci S, Scharfmann R. Fgf10 is essential for maintaining the proliferative capacity of epithelial progenitor cells during early pancreatic organogenesis. Development. 2001. 128:5109–5117.


6. Gu G, Dubauskaite J, Melton DA. Direct evidence for the pancreatic lineage. NGN3+ cells are islet progenitors and are distinct from duct progenitors. Development. 2002. 129:2447–2457.


7. Ianus A, Holz GG, Theise ND, Hussain MA. in vivo derivation of glucose-competent pancreatic endocrine cells from bone marrow without evidence of cell fusion. J Clin Invest. 2003. 111:843–850.


8. Hess D, Li L, Martin M, Sakano S, Hill D, Strutt B, Thyssen S, Gray DA, Bhatia M. Bone marrow-derived stem cells initiate pancreatic regeneration. Nat Biotechnol. 2003. 21:763–770.


9. Taneera J, Rosengren A, Renstrom E, Nygren JM, Serup P, Rorsman P, Jacobsen SE. Failure of transplanted bone marrow cells to adopt a pancreatic beta-cell fate. Diabetes. 2006. 55:290–296.


10. Tang DQ, Cao LZ, Burkhardt BR, Xia CQ, Litherland SA, Atkinson MA, Yang LJ. in vivo and in vitro characterization of insulin-producing cells obtained from murine bone marrow. Diabetes. 2004. 53:1721–1732.


11. Oh SH, Muzzonigro TM, Bae SH, LaPlante JM, Hatch HM, Petersen BE. Adult bone marrow-derived cells trans-differentiating into insulin-producing cells for the treatment of type I diabetes. Lab Invest. 2004. 84:607–617.


12. Lee RH, Seo MJ, Reger RL, Spees JL, Pulin AA, Olson SD, Prockop DJ. Multipotent stromal cells from human marrow home to and promote repair of pancreatic islets and renal glomeruli in diabetic NOD/scid mice. Proc Natl Acad Sci U S A. 2006. 103:17438–17443.


13. Karnieli O, Izhar-Prato Y, Bulvik S, Efrat S. Generation of insulin-producing cells from human bone marrow mesenchymal stem cells by genetic manipulation. Stem Cells. 2007. 25:2837–2844.


14. Luo L, Luo JZ, Xiong F, Abedi M, Greer D. Cytokines inducing bone marrow SCA+ cells migration into pancreatic islet and conversion into insulin-positive cells in vivo. PLoS One. 2009. 4:e4504.
15. Timper K, Seboek D, Eberhardt M, Linscheid P, Christ-Crain M, Keller U, Müller B, Zulewski H. Human adipose tissue-derived mesenchymal stem cells differentiate into insulin, somatostatin, and glucagon expressing cells. Biochem Biophys Res Commun. 2006. 341:1135–1140.


16. Trivedi HL, Vanikar AV, Thakker U, Firoze A, Dave SD, Patel CN, Patel JV, Bhargava AB, Shankar V. Human adipose tissue-derived mesenchymal stem cells combined with hematopoietic stem cell transplantation synthesize insulin. Transplant Proc. 2008. 40:1135–1139.


17. Chandra V, G S, Phadnis S, Nair PD, Bhonde RR. Generation of pancreatic hormone-expressing islet-like cell aggregates from murine adipose tissue-derived stem cells. Stem Cells. 2009. 27:1941–1953.


18. Kang HM, Kim J, Park S, Kim H, Kim KS, Lee EJ, Seo SI, Kang SG, Lee JE, Lim H. Insulin-secreting cells from human eyelid-derived stem cells alleviate type I diabetes in immunocompetent mice. Stem Cells. 2009. 27:1999–2008.


19. Lin G, Wang G, Liu G, Yang L, Chang LJ, Lue TF, Lin CS. Treatment of type 1 diabetes with adipose tissue-derived stem cells expressing pancreatic duodenal homeobox 1. Stem Cells Dev. 2009. in press.


20. Sun B, Roh KH, Lee SR, Lee YS, Kang KS. Induction of human umbilical cord blood-derived stem cells with embryonic stem cell phenotypes into insulin producing islet-like structure. Biochem Biophys Res Commun. 2007. 354:919–923.


21. Denner L, Bodenburg Y, Zhao JG, Howe M, Cappo J, Tilton RG, Copland JA, Forraz N, McGuckin C, Urban R. Directed engineering of umbilical cord blood stem cells to produce C-peptide and insulin. Cell Prolif. 2007. 40:367–380.


22. Gao F, Wu DQ, Hu YH, Jin GX, Li GD, Sun TW, Li FJ. in vitro cultivation of islet-like cell clusters from human umbilical cord blood-derived mesenchymal stem cells. Transl Res. 2008. 151:293–302.


23. Yoshida S, Ishikawa F, Kawano N, Shimoda K, Nagafuchi S, Shimoda S, Yasukawa M, Kanemaru T, Ishibashi H, Shultz LD, Harada M. Human cord blood--derived cells generate insulin-producing cells in vivo. Stem Cells. 2005. 23:1409–1416.


24. Jin CX, Li WL, Xu F, Geng ZH, He ZY, Su J, Tao XR, Ding XY, Wang X, Hu YP. Conversion of immortal liver progenitor cells into pancreatic endocrine progenitor cells by persistent expression of Pdx-1. J Cell Biochem. 2008. 104:224–236.


25. Ferber S, Halkin A, Cohen H, Ber I, Einav Y, Goldberg I, Barshack I, Seijffers R, Kopolovic J, Kaiser N, Karasik A. Pancreatic and duodenal homeobox gene 1 induces expression of insulin genes in liver and ameliorates streptozotocin-induced hyperglycemia. Nat Med. 2000. 6:568–572.


26. Kaneto H, Nakatani Y, Miyatsuka T, Matsuoka TA, Matsuhisa M, Hori M, Yamasaki Y. PDX-1/VP16 fusion protein, together with NeuroD or Ngn3, markedly induces insulin gene transcription and ameliorates glucose tolerance. Diabetes. 2005. 54:1009–1022.


27. Yang L, Li S, Hatch H, Ahrens K, Cornelius JG, Petersen BE, Peck AB. in vitro trans-differentiation of adult hepatic stem cells into pancreatic endocrine hormone-producing cells. Proc Natl Acad Sci U S A. 2002. 99:8078–8083.


28. Zalzman M, Gupta S, Giri RK, Berkovich I, Sappal BS, Karnieli O, Zern MA, Fleischer N, Efrat S. Reversal of hyperglycemia in mice by using human expandable insulin-producing cells differentiated from fetal liver progenitor cells. Proc Natl Acad Sci U S A. 2003. 100:7253–7258.


29. Meier JJ, Bhushan A, Butler AE, Rizza RA, Butler PC. Sustained beta cell apoptosis in patients with long-standing type 1 diabetes: indirect evidence for islet regeneration? Diabetologia. 2005. 48:2221–2228.


30. Bonner-Weir S, Baxter LA, Schuppin GT, Smith FE. A second pathway for regeneration of adult exocrine and endocrine pancreas. A possible recapitulation of embryonic development. Diabetes. 1993. 42:1715–1720.


31. Ramiya VK, Maraist M, Arfors KE, Schatz DA, Peck AB, Cornelius JG. Reversal of insulin-dependent diabetes using islets generated in vitro from pancreatic stem cells. Nat Med. 2000. 6:278–282.


32. Bonner-Weir S, Taneja M, Weir GC, Tatarkiewicz K, Song KH, Sharma A, O’Neil JJ. in vitro cultivation of human islets from expanded ductal tissue. Proc Natl Acad Sci USA. 2000. 97:7999–8004.
33. Rooman I, Bouwens L. Combined gastrin and epidermal growth factor treatment induces islet regeneration and restores normoglycaemia in C57Bl6/J mice treated with alloxan. Diabetologia. 2004. 47:259–265.


34. Hao E, Tyrberg B, Itkin-Ansari P, Lakey JR, Geron I, Monosov EZ, Barcova M, Mercola M, Levine F. Beta-cell differentiation from nonendocrine epithelial cells of the adult human pancreas. Nat Med. 2006. 12:310–316.


35. Xu X, D’Hoker J, Stangé G, Bonne S, De Leu N, Xiao X, Van De Casteele M, Mellitzer G, Ling Z, Pipeleers D, Bouwens L, Scharfmann R, Gradwohl G, Heimberg H. Beta cells can be generated from endogenous progenitors in injured adult mouse pancreas. Cell. 2008. 132:197–207.


36. Seaberg RM, Smukler SR, Kieffer TJ, Enikolopov G, Asghar Z, Wheeler MB, Korbutt G, van der Kooy D. Clonal identification of multipotent precursors from adult mouse pancreas that generate neural and pancreatic lineages. Nat Biotechnol. 2004. 22:1115–1124.


37. Lechner A, Nolan AL, Blacken RA, Habener JF. Redifferentiation of insulin-secreting cells after in vitro expansion of adult human pancreatic islet tissue. Biochem Biophys Res Commun. 2005. 327:581–588.


38. Chase LG, Ulloa-Montoya F, Kidder BL, Verfaillie CM. Islet-derived fibroblast-like cells are not derived via epithelial-mesenchymal transition from Pdx-1 or insulin-positive cells. Diabetes. 2007. 56:3–7.


Fig. 1.
Protocols used for differentiation of adipocyte-derived stem cells into insulin-producing cells. Human or mouse adipose tissue-derived stem cells (ADSCs) and human eyelid adipose tissue-derived stem cells (HEACs) were used for differentiation. The factors used for differentiation at each stage, and in vitro characterization and outcomes of in vivo functional tests of the differentiated insulin-producing cells are denoted. DMEM: dulbecco’s modified eagle’ medium, HGF: hepatocyte growth factor, FBS: fetal bovine serum, GLP-1: glucagon-like peptide, BSA: bovine serum albumin, ITS: insulin-transferrin-selenium, Sod. butyrate: sodium butyrate, 2-ME: 2-mercaptoethanol, NEAA: non essential amino acids
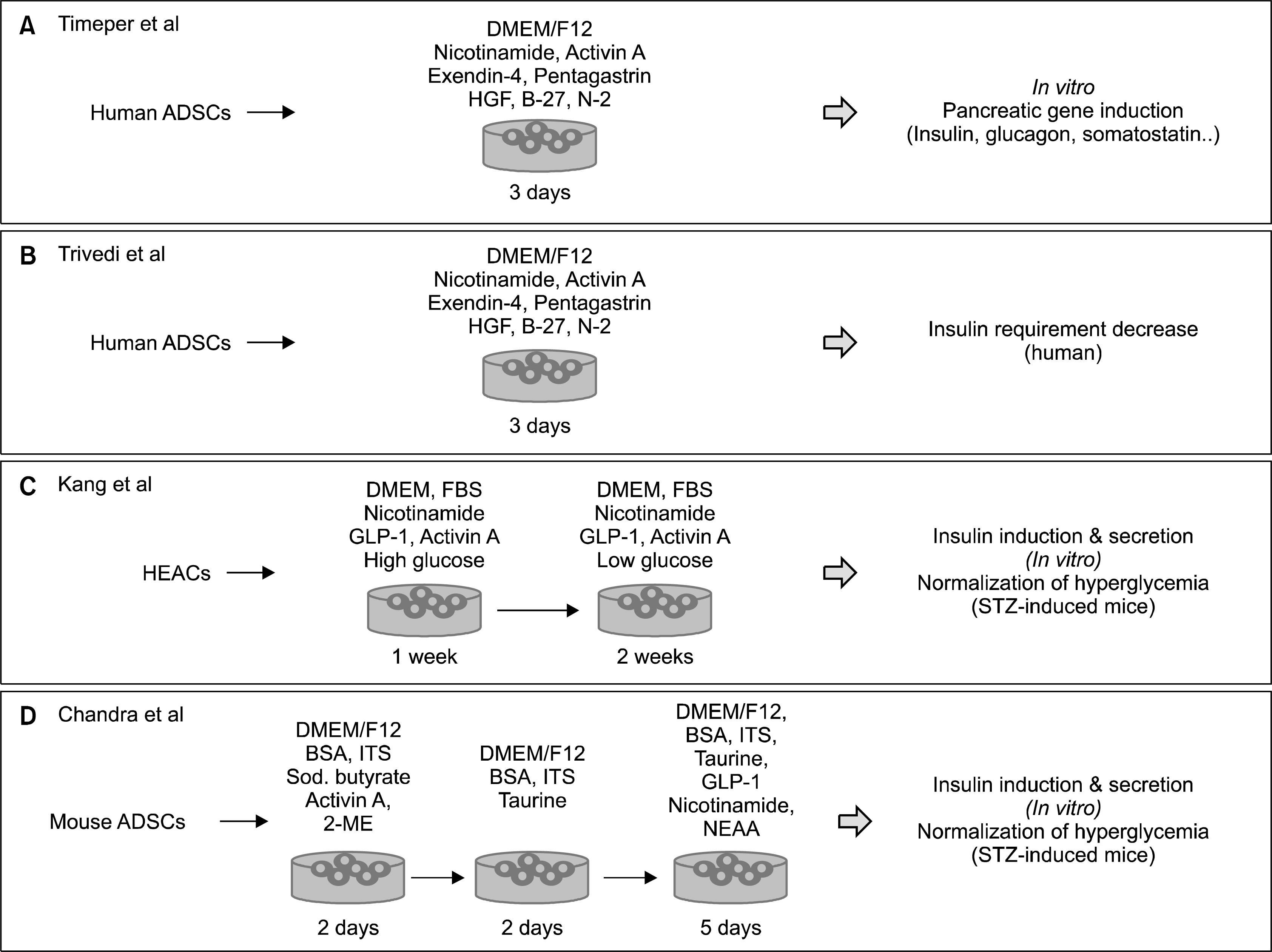