Abstract
Background
There are conflicting opinions on the effect of dexmedetomidine on cerebral autoregulation. This study assessed its effect on dynamic cerebral autoregulation (dCA) using a transcranial Doppler (TCD).
Methods
Thirty American Society of Anesthesiologists physical status I and II patients between 18 and 60 years, who underwent lumbar spine surgery, received infusions of dexmedetomidine (Group D) or normal saline (Group C), followed by anesthesia with propofol and fentanyl, and maintenance with oxygen, nitrous oxide and sevoflurane. After five minutes of normocapnic ventilation and stable bispectral index value (BIS) of 40–50, the right middle cerebral artery flow velocity (MCAFV) was recorded with TCD. The transient hyperemic response (THR) test was performed by compressing the right common carotid artery for 5–7 seconds. The lungs were hyperventilated to test carbon dioxide (CO2) reactivity. Hemodynamic parameters, arterial CO2 tension, pulse oximetry (SpO2), MCAFV and BIS were measured before and after hyperventilation. Dexmedetomidine infusion was discontinued ten minutes before skin-closure. Time to recovery and extubation, modified Aldrete score, and emergence agitation were recorded.
Results
Demographic parameters, durations of surgery and anesthesia, THR ratio (Group D: 1.26 ± 0.11 vs. Group C: 1.23 ± 0.04; P = 0.357), relative CO2 reactivity (Group D: 1.19 ± 0.34 %/mmHg vs. Group C: 1.23 ± 0.25 %/mmHg; P = 0.547), blood pressure, SpO2, BIS, MCAFV, time to recovery, time to extubation and modified Aldrete scores were comparable.
Cerebral autoregulation describes the intrinsic ability of the cerebral vasculature to maintain a stable cerebral blood flow (CBF) over a wide range of arterial blood pressures. Preservation of cerebral autoregulation is an important goal in anesthetized patients. Apart from pathological conditions, impairment of autoregulation under anesthesia may result from anesthetic drug-related factors and various physiological variables such as carbon dioxide changes and anemia from hemodilution [1,2]. Volatile agents, with the possible exception of sevoflurane, have a dose-related depressive effect on cerebral autoregulation [3]. Sedatives, hypnotics, and analgesics may induce changes in CBF by altering cerebral autoregulation [4]. Injudicious use of these anesthetic agents may aggravate pre-existing intracranial pathology and yield poor postoperative outcomes. It is imperative to understand the effects of various anesthetic agents on cerebral autoregulation during physiological and pathological states.
Dexmedetomidine is a highly selective alpha2 adrenergic agonist used in clinical anesthesia for sedation, analgesia, sympatholysis and anesthesia-sparing properties [5]. It facilitates good perioperative hemodynamic stability and reduces intraoperative opioid requirement [6]. Unlike other commonly used anesthetic drugs, dexmedetomidine does not cause respiratory depression. This is beneficial in surgeries that require intraoperative patient cooperation, such as awake craniotomy and carotid endarterectomy. Various studies have suggested the neuroprotective role of dexmedetomidine, making it a suitable adjuvant to neurosurgical anesthesia [6]. It also lowers CBF, which may be of benefit in intracranial surgical procedures [7,8]. The effect of dexmedetomidine on cerebral autoregulation and carbon dioxide reactivity under anesthesia in humans have not been studied.
The primary aim of our study was to assess the effect of dexmedetomidine on cerebral autoregulation and carbon dioxide reactivity under sevoflurane anesthesia in patients undergoing spine surgery. The secondary objectives were to assess its effects on intraoperative hemodynamic parameters, requirement of other anesthetic agents, time to tracheal extubation and recovery, quality of emergence and complications.
Ethical approval for this study was obtained from the Institute Ethics Committee/ Ethics Sub-Committee, All India Institute of Medical Sciences, New Delhi, India (Approval number IESC/T-369/30.08.2013 dated 04.11.2013). The trial was registered in the clinical trials registry (CTRI/2015/06/005875). Written informed consent was obtained from all the patients. The sample size was chosen as a convenient feasible number based on the resources available for the conduct of this preliminary study. Thirty consecutive American Society of Anesthesiologists physical status I and II patients, between the ages of 18 and 60 years, who underwent elective lumbar spine surgery in our hospital were enrolled in the study. Patients with history of syncope, dizziness, cardiac or liver diseases were excluded from the study. Preoperative carotid Doppler was performed in all patients to rule out carotid pathologies. On the day of surgery, analgesic treatments, if any, were discontinued. In the operating room, electrocardiogram, pulse oximeter (SpO2), non-invasive blood pressure and bispectral index (BIS) sensor (Datex-Ohmeda, USA) were attached. The radial artery was cannulated and a sample for blood gases was obtained before the induction of general anesthesia.
The patients were assigned to one of two 15-member groups, using a computer-generated randomization chart. The random numbers were placed serially in consecutively labelled, opaque envelopes. Patients in the study group (Group D) received 1 μg/kg bolus (4 μg/ml concentration) intravenous infusions of dexmedetomidine over 10 minutes, followed by 0.5 μg/kg/h as maintenance throughout the surgical procedure. Patients in the control group (Group C) received normal saline infusion at identical rates through a similar syringe-infusion pump, presuming that the infusate contained dexmedetomidine. An anesthesiologist, who was part of this study, but not involved in patient management, prepared the infusions in 50 ml syringes of the same make. The anesthesiologist (SB) performing transcranial Doppler (TCD) measurements was blinded to the nature of the content in the syringe. After infusion of the bolus dose, anesthesia was induced with propofol 1–2 mg/kg and fentanyl 2 µg/kg. Tracheal intubation was facilitated using vecuronium 0.1 mg/kg. Anesthesia was maintained using oxygen with nitrous oxide (1 : 1) and sevoflurane, adjusted to maintain BIS value between 40 and 50. Further boluses of fentanyl and vecuronium were administered at intermittent intervals based on the clinical judgment of the consultant anesthesiologist.
After five minutes of sustained BIS within the pre-defined range and normocapnia (end-tidal carbon dioxide between 35–40 mmHg), a 2-MHz frequency hand-held TCD probe (Digi-Lite, Rimed Ltd., USA) was placed on the right temporal window above the zygomatic arch of the patient in supine position, and the right middle cerebral artery (MCA) was insonated. The MCA was identified through the transtemporal window after obtaining a strong, consistent spectral signal with flow direction towards the probe (upward deflection) at a depth of 40–60 mm. Transient hyperemic response (THR) test, which is a measure of cerebral autoregulation, was performed using the following steps. Middle cerebral artery flow velocity (MCAFV) at baseline (F1) was recorded, and the ipsilateral carotid artery was compressed by an assistant for 5–7 seconds. The MCAFV during compression (F2) was noted. The carotid compression was released and MCAFV was noted immediately for a second time (F3). This test was repeated thrice, and the recorded values were averaged for final analysis. The test was accepted only if (a) compression of the carotid artery led to a sudden and maximal decrease in MCAFV (no further decrease in MCAFV could be achieved), (b) heart rate (HR) and mean arterial pressure (MAP) remained stable during compression (within 10% of their respective pre-compression values), (c) no flow transients were seen in the Doppler signal after release of the carotid compression, and (d) power of the reflected Doppler signal remained unchanged during the test. The THR test was chosen because it is simple and quick to perform, non-invasive and has been well-validated as a measure of dynamic cerebral autoregulation. Finally, the THR Ratio (THRR) was calculated using the formula given by Czosnyka: THRR = F3 / F1 [9]. A ratio above 1.10 was considered as intact dCA, while values lower than 1.10 were interpreted as poor or absent autoregulation. To measure the magnitude of decrease in blood flow velocity during carotid compression, the compression ratio (CR) was calculated using the formula given by Smielewski et al. [10]: CR (%) = (F1 – F2) x 100 / F1. Since carbon dioxide (CO2) reactivity may remain intact even when autoregulation is lost, we analyzed arterial blood gases at baseline and after hyperventilation to reduce the end-tidal CO2 (EtCO2) by 8–10 mmHg, while testing for autoregulation. A minimum of 5 minutes for stabilization (unchanged EtCO2) was allowed at each PaCO2 level before measurements were made. Three pairs of PaCO2-MCAFV data were obtained in each patient for determination of CO2 reactivity.
Absolute CO2 reactivity, defined as the change in MCAFV per mmHg change in PaCO2 and Relative CO2 reactivity, defined as the percent change in MCAFV per mmHg decrease in PaCO2, were calculated using the following formulas:
Absolute CO2 reactivity = (Initial MCAFV – Final MCAFV) / (Initial PaCO2 – Final PaCO2)
Relative CO2 reactivity = (Absolute CO2 reactivity / Initial MCAFV) × 100
(Initial MCAFV, Final MCAFV are MCAFV before and after hyperventilation, and initial and final PaCO2 are arterial CO2 tensions before and after hyperventilation).
The tests were accepted only if no hemodynamic changes were observed during hyperventilation (HR and MAP remained within 10% of baseline values).
After these tests, surgery was carried out as planned. The infusion of the test drug or placebo was discontinued 10 minutes before skin closure. The trachea was extubated after reversing residual neuromuscular blockade with neostigmine and glycopyrrolate, and the patient was fully awake. Time to recovery, time to extubation, quality of emergence and complications during the procedure were recorded. ‘Time to recovery’ was defined as the time after turning off the anesthetic gases until the patient followed commands. ‘Time to extubation’ was defined as the time from stopping the anesthetic gases until tracheal extubation. The presence of emergence agitation was considered as poor recovery while its absence was classified as good recovery. A modified Aldrete score of less than 9 was considered as poor recovery. Any complication during the perioperative period was recorded.
Data were represented as mean ± SD. Continuous data were compared by using student t-test or Mann-Whitney test, whereas qualitative data were analyzed using Chi-square test or Fischer exact test. A P value of less than 0.05 was considered as significant.
The demographic profile was comparable between groups (Table 1). There were no procedural difficulties in insonating the temporal window for conducting TCD or performing the THR test in any patient (Fig. 1). The cerebral autoregulation indices measured by TCD such as THRR (P = 0.357), CR (P = 0.158), absolute CO2 reactivity (P = 0.618), and relative CO2 reactivity (P = 0.547) were comparable in both groups (Table 2). MCAFV was also comparable between the two groups before hyperventilation (61 ± 9 in group D vs. 64 ± 8 cm/s in group C; P = 0.340) and after hyperventilation (56 ± 9 in Group D vs. 57 ± 10 cm/s Group C; P = 0.650, Fig. 2). A significant but comparable decrease in PaCO2 levels was achieved in both groups before hyperventilation (38 ± 2 mmHg in Group D, vs. 39 ± 2 mmHg in Group C; P = 0.160), and after hyperventilation (29 ± 2 mmHg in Group D vs. 30 ± 2 mmHg in Group C, P = 0.240, Fig. 2). Volume of fluids infused and urine output in both groups were comparable. However, there was a significant difference in intraoperative blood loss between the two groups (Table 1).
There was no difference in propofol consumption between groups. Median durations of surgery and anesthesia were also comparable. The total doses of fentanyl and vecuronium used were significantly lower in Group D than in Group C. The depth of anesthesia was comparable between groups before and after hyperventilation. Blood pressure before and after hyperventilation in both groups was comparable (Fig. 3). HR was significantly lower in Group D before and after hyperventilation.
Times to tracheal extubation and recovery, and modified Aldrete score were similar between groups (Table 3). One patient in Group C had emergence agitation (modified Aldrete score 8; trachea was re-intubated for two hours). No other side effect or complication was observed.
In our study, we did not observe any adverse effects of dexmedetomidine on cerebral autoregulation and carbon dioxide reactivity under sevoflurane anesthesia. One previous animal-based study found that dexmedetomidine decreases CBF due to a combination of direct cerebral vasoconstriction, depressed cerebral metabolism and reduction in systemic blood pressure from its administration [11]. However, this study was conducted using halothane anesthesia in rats using extremely high doses of dexmedetomidine that are never used in clinical anesthesia. Furthermore, the study was based on cerebral microcirculation and not on large vessels like MCA. To the best of our knowledge, there are no large human studies assessing the effects of dexmedetomidine on cerebral autoregulation under the effect of general anesthesia. Previous studies on the effects of dexmedetomidine on cerebral autoregulation have been confined to small populations of awake patients, with or without brain pathology. Ogawa et al. [8], from their study on healthy volunteers, concluded that dexmedetomidine weakens dynamic cerebral autoregulation and delays the restoration of CBF velocity. However, this was a small cross-over study using various doses of dexmedetomidine in only 14 awake volunteers. They also used transfer function analysis to study cerebral autoregulation. Another recently published small study on 15 awake patients with supratentorial tumors, by Arulvelan et al. [12], concluded that with a bolus dose of 1 µg/kg of dexmedetomidine, there was no change in autoregulatory indices in the hemisphere bearing the tumor, but the indices were deranged in the non-pathologic hemisphere.
THR test, also called carotid compression test, was first described by Giller [13], as a bedside test for the assessment of cerebral autoregulation on the premise that if autoregulation is effective, the reduction in MCA pressure from common carotid artery compression should lead to reflex vasodilatation in the arterioles distal to the MCA. Therefore, release of compression would result in a transient increase in CBF, and the MCA flow velocity. This would be observed as increased flow velocity in TCD tests (Fig. 4). This hyperemic response is absent when autoregulation is lost [14]. This test is simple, non-invasive and can be repeated without much discomfort to the patient. Several studies have validated this concept in animal models and in humans with conditions like traumatic brain injury and subarachnoid hemorrhage [14–16]. Smielewski et al. [10], have further shown that THRR is a valid index of cerebral autoregulation and has similar sensitivity to the leg-cuff method described by Aaslid et al. [10,17]. A THRR more than 1.10 implies intact autoregulation, while values below 1.10 suggest poor or absent autoregulation [10]. The CR, which measures the magnitude of carotid compression, was similar in both groups.
No previous study has evaluated the effects of dexmedetomidine on carbon dioxide reactivity. TCD has proven to be a useful point-of-care tool to determine CO2 reactivity compared to conventional direct methods of CBF measurements [18]. We did not observe any influence of dexmedetomidine on absolute and relative CO2 reactivity. This observation is consistent with previous studies that have demonstrated that carbon dioxide reactivity of cerebral vessels is very robust and remains intact even in some cerebral pathological states. Cerebrovascular CO2 reactivity under sevoflurane anesthesia has been shown to be lower in patients with previous stroke [19]. Kirkham et al. [20] have demonstrated that CO2 reactivity determined by TCD correlates well with CO2 reactivity determined using direct methods of measuring CBF. Measuring CO2 reactivity and cerebral autoregulation in the initial phase of anesthesia keeps body temperature and hematocrit levels largely unchanged. During neurosurgery, hematocrit levels may fluctuate with bleeding and blood transfusion, and alter blood viscosity and cerebral blood flow velocity [21].
Theoretically, the difference in sevoflurane concentration could have affected autoregulation in the two groups. However, only a few isolated studies have indicated that cerebral autoregulation may be affected by sevoflurane anesthesia [22]. Since we completed our TCD measurements during the initial phase of anesthesia and before the start of surgery, it is very unlikely that autoregulation was influenced to any significant extent by sevoflurane. Several human studies suggest that sevoflurane in concentrations less than 1–1.2 MAC does not disrupt cerebral autoregulation or affect cerebrovascular reactivity to carbon dioxide [23–27]. Similar observations have also been made in young children [28,29], and when nitrous oxide is used along with sevoflurane [30]. A study in patients with diabetes found that sevoflurane-based anesthesia does not affect cerebral autoregulation when it is already impaired [31].
Carotid manipulation in the neck is a seemingly innocuous procedure, but may sometimes result in serious complications [32]. In our study, preoperative carotid Doppler study of all patients was conducted to rule out any atheromatous plaque of the artery. We also excluded elderly patients, who have higher risks of atheromatous plaques in their arteries. It has not been reported in literature that brain insult may occur following a brief carotid compression, as part of the THR test, in the absence of carotid pathology. Even though hypotension and bradycardia may occur because of carotid compression, no such complication was encountered in our study. The actual drop in cerebral perfusion pressure (CPP) during compression is not known and may depend on the magnitude of force, the duration of compression and the effectiveness of the collateral blood supply. Although these concerns do not appear to be of practical importance in healthy individuals, patients with deranged intracranial compliance may experience increase in intracranial pressure and thus, decrease in CPP during this procedure.
Dexmedetomidine use was not associated with any delay in offset of anesthesia in this study. This may be due its opioid- and anesthetic-sparing effects. The use of BIS, with pre-set target values, may have also helped to prevent delay in recovery from anesthesia. BIS monitoring has been shown to reduce anesthetic consumption [33].
From our results, we infer that dexmedetomidine administration in clinical doses under sevoflurane-based anesthesia does not weaken dynamic cerebral autoregulation or carbon dioxide reactivity in patients without concomitant brain pathology. These observations are similar to those of Drummond et al. [7], who reported that dexmedetomidine administration in healthy and awake volunteers preserves carbon dioxide reactivity. However, their observations cannot be extrapolated to patients who are under the effect of general anesthesia. They also did not study the effect of dexmedetomidine on dynamic cerebral autoregulation.
A major limitation of our study is that it was conducted in healthy adult patients without any intracranial pathology and therefore, our observations cannot be extrapolated to all patients, such as those with pre-existing cerebral pathology. Even though nitrous oxide was used in both groups of patients, and a stable BIS value was always ensured, we cannot be certain that the study results were not influenced in any way by the effect of nitrous oxide. Due to logistical reasons, the sample size of the study was restricted to 30 patients. The findings of this study can provide the foundation for designing a large randomized trial for possible generalization of these findings across all populations of neurosurgical patients.
In conclusion, dexmedetomidine administration, in standard doses as an anesthetic adjuvant under sevoflurane anesthesia, does not impair dynamic cerebral autoregulation and carbon dioxide reactivity in the absence of intracranial pathology. However, further studies are required to fully ascertain the effect of dexmedetomidine in different age groups of anesthetized patients with various kinds of intracranial pathologies.
Notes
Author Contributions
Sujoy Banik (Data curation; Formal analysis; Investigation; Methodology; Writing – original draft)
Girija Prasad Rath (Conceptualization; Data curation; Investigation; Methodology; Supervision; Writing – review & editing)
Ritesh Lamsal (Formal analysis; Investigation; Validation; Writing – original draft; Writing – review & editing)
Parmod K Bithal (Methodology; Supervision; Validation; Writing – original draft; Writing – review & editing)
References
1. Ainslie PN, Celi L, McGrattan K, Peebles K, Ogoh S. Dynamic cerebral autoregulation and baroreflex sensitivity during modest and severe step changes in arterial PCO2. Brain Res. 2008; 1230:115–24.
2. Todd MM. Cerebral blood flow during isovolemic hemodilution: mechanistic observations. Adv Pharmacol. 1994; 31:595–605.


3. Dagal A, Lam AM. Cerebral autoregulation and anesthesia. Curr Opin Anaesthesiol. 2009; 22:547–52.


4. Grathwohl KW, Black IH, Spinella PC, Sweeney J, Robalino J, Helminiak J, et al. Total intravenous anesthesia including ketamine versus volatile gas anesthesia for combat-related operative traumatic brain injury. Anesthesiology. 2008; 109:44–53.


5. Keniya VM, Ladi S, Naphade R. Dexmedetomidine attenuates sympathoadrenal response to tracheal intubation and reduces perioperative anaesthetic requirement. Indian J Anaesth. 2011; 55:352–7.


6. Tanskanen PE, Kytta JV, Randell TT, Aantaa RE. Dexmedetomidine as an anaesthetic adjuvant in patients undergoing intracranial tumour surgery: a double-blind, randomized and placebo-controlled study. Br J Anaesth. 2006; 97:658–65.
7. Drummond JC, Dao AV, Roth DM, Cheng CR, Atwater BI, Minokadeh A, et al. Effect of dexmedetomidine on cerebral blood flow velocity, cerebral metabolic rate, and carbon dioxide response in normal humans. Anesthesiology. 2008; 108:225–32.


8. Ogawa Y, Iwasaki K, Aoki K, Kojima W, Kato J, Ogawa S. Dexmedetomidine weakens dynamic cerebral autoregulation as assessed by transfer function analysis and the thigh cuff method. Anesthesiology. 2008; 109:642–50.


9. Czosnyka M, Pickard J, Whitehouse H, Piechnik S. The hyperaemic response to a transient reduction in cerebral perfusion pressure. A modelling study. Acta Neurochir (Wien). 1992; 115:90–7.
10. Smielewski P, Czosnyka M, Kirkpatrick P, McEroy H, Rutkowska H, Pickard JD. Assessment of cerebral autoregulation using carotid artery compression. Stroke. 1996; 27:2197–203.


11. Ganjoo P, Farber NE, Hudetz A, Smith JJ, Samso E, Kampine JP, et al. In vivo effects of dexmedetomidine on laser-Doppler flow and pial arteriolar diameter. Anesthesiology. 1998; 88:429–39.


12. Arulvelan A, Manikandan S, Easwer HV, Krishnakumar K. Effect of loading dose of dexmedetomidine on dynamic cerebral blood flow autoregulation in patients with intracranial glial neoplasms. J Neurosurg Anesthesiol. 2015; 27:289–94.


13. Giller CA. A bedside test for cerebral autoregulation using transcranial Doppler ultrasound. Acta Neurochir (Wien). 1991; 108:7–14.


14. Smielewski P, Czosnyka M, Kirkpatrick P, Pickard JD. Evaluation of the transient hyperemic response test in head-injured patients. J Neurosurg. 1997; 86:773–8.


15. Lam JM, Smielewski P, Czosnyka M, Pickard JD, Kirkpatrick PJ. Predicting delayed ischemic deficits after aneurysmal subarachnoid hemorrhage using a transient hyperemic response test of cerebral autoregulation. Neurosurgery. 2000; 47:819–25. ; discussions 25-6.


16. Lang EW, Mehdorn HM, Dorsch NW, Czosnyka M. Continuous monitoring of cerebrovascular autoregulation: a validation study. J Neurol Neurosurg Psychiatry. 2002; 72:583–6.


17. Aaslid R, Lindegaard KF, Sorteberg W, Nornes H. Cerebral autoregulation dynamics in humans. Stroke. 1989; 20:45–52.


18. Hirst RP, Slee TA, Lam AM. Changes in cerebral blood flow velocity after release of intraoperative tourniquets in humans: a transcranial Doppler study. Anesth Analg. 1990; 71:503–10.
19. Kadoi Y, Saito S, Takahashi K. The comparative effects of sevoflurane versus isoflurane on cerebrovascular carbon dioxide reactivity in patients with previous stroke. J Anesth. 2008; 22:135–9.


20. Kirkham FJ, Padayachee TS, Parsons S, Seargeant LS, House FR, Gosling RG. Transcranial measurement of blood velocities in the basal cerebral arteries using pulsed Doppler ultrasound: velocity as an index of flow. Ultrasound Med Biol. 1986; 12:15–21.


21. Brass LM, Pavlakis SG, DeVivo D, Piomelli S, Mohr JP. Transcranial Doppler measurements of the middle cerebral artery. Effect of hematocrit. Stroke. 1988; 19:1466–9.


22. Ogawa Y, Iwasaki K, Shibata S, Kato J, Ogawa S, Oi Y. The effect of sevoflurane on dynamic cerebral blood flow autoregulation assessed by spectral and transfer function analysis. Anesth Analg. 2006; 102:552–9.


23. Summors AC, Gupta AK, Matta BF. Dynamic cerebral autoregulation during sevoflurane anesthesia: a comparison with isoflurane. Anesth Analg. 1999; 88:341–5.
24. Gupta S, Heath K, Matta BF. Effect of incremental doses of sevoflurane on cerebral pressure autoregulation in humans. Br J Anaesth. 1997; 79:469–72.


25. Molnár C, Settakis G, Sárkány P, Kálmán S, Szabó S, Fülesdi B. Effect of sevoflurane on cerebral blood flow and cerebrovascular resistance at surgical level of anaesthesia: a transcranial Doppler study. Eur J Anaesthesiol. 2007; 24:179–84.


26. Rozet I, Vavilala MS, Lindley AM, Visco E, Treggiari M, Lam AM. Cerebral autoregulation and CO2 reactivity in anterior and posterior cerebral circulation during sevoflurane anesthesia. Anesth Analg. 2006; 102:560–4.
27. Juhasz M, Molnar L, Fulesdi B, Vegh T, Pall D, Molnar C. Effect of sevoflurane on systemic and cerebral circulation, cerebral autoregulation and CO2 reactivity. BMC Anesthesiol. 2019; 19:109.


28. Wong GT, Luginbuehl I, Karsli C, Bissonnette B. The effect of sevoflurane on cerebral autoregulation in young children as assessed by the transient hyperemic response. Anesth Analg. 2006; 102:1051–5.


29. Vavilala MS, Lee LA, Lee M, Graham A, Visco E, Lam AM. Cerebral autoregulation in children during sevoflurane anaesthesia. Br J Anaesth. 2003; 90:636–41.
30. Cho S, Fujigaki T, Uchiyama Y, Fukusaki M, Shibata O, Sumikawa K. Effects of sevoflurane with and without nitrous oxide on human cerebral circulationTranscranial doppler study. Anesthesiology. 1996; 85:755–60.
31. Sperna Weiland NH, Hermanides J, van der Ster BJ, Hollmann MW, Preckel B, Stok WJ, et al. Sevoflurane based anaesthesia does not affect already impaired cerebral autoregulation in patients with type 2 diabetes mellitus. Br J Anaesth. 2018; 121:1298–307.


33. Karwacki Z, Niewiadomski S, Rzaska M, Witkowska M. The effect of bispectral index monitoring on anaesthetic requirements in target-controlled infusion for lumbar microdiscectomy. Anaesthesiol Intensive Ther. 2014; 46:284–8.
Fig. 2.
Comparison of partial pressure of carbon dioxide and mid-brain artery flow rates in two groups before and after hyperventilation. Group D: dexmedetomidine administration, Group C: normal saline administration. PaCO2: partial pressure of arterial carbon dioxide, MCAFV: middle cerebral artery flow rate.
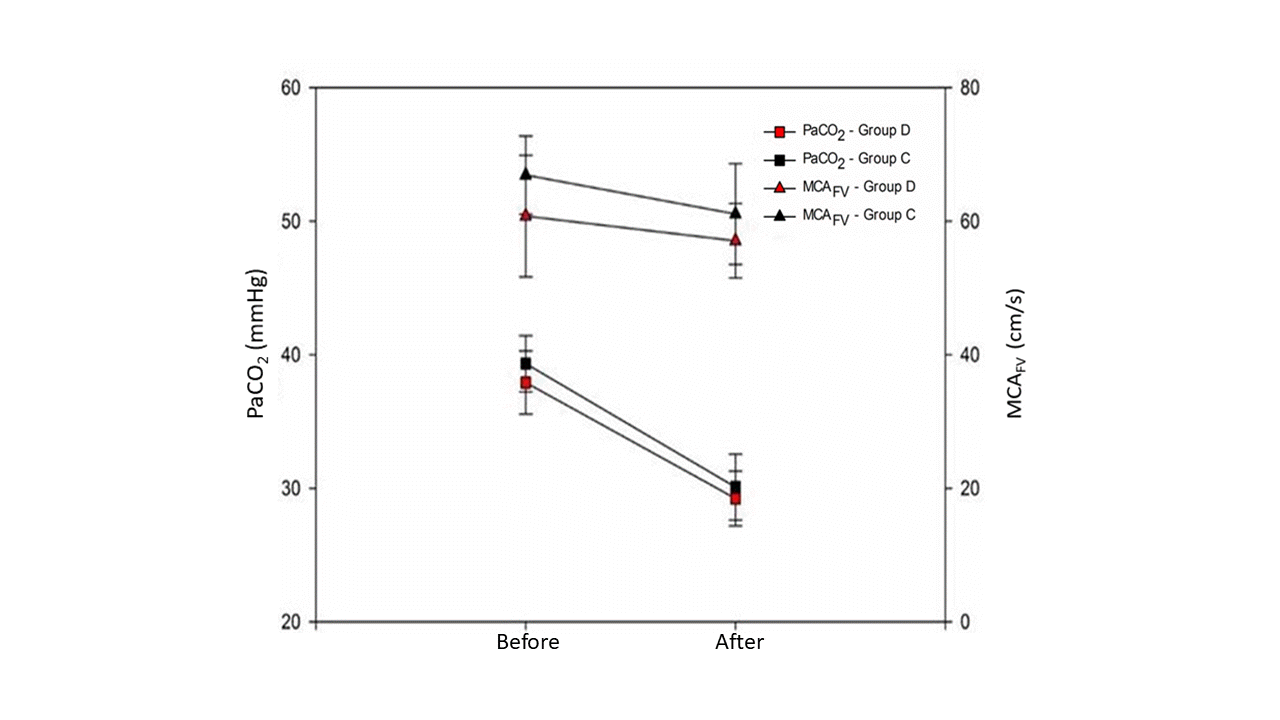
Fig. 3.
Comparison of heart rate and average arterial pressure before and after hyperventilation. Group D: dexmedetomidine administration. Group C: normal saline administration. HR: heart rate, MAP: mean arterial pressure.
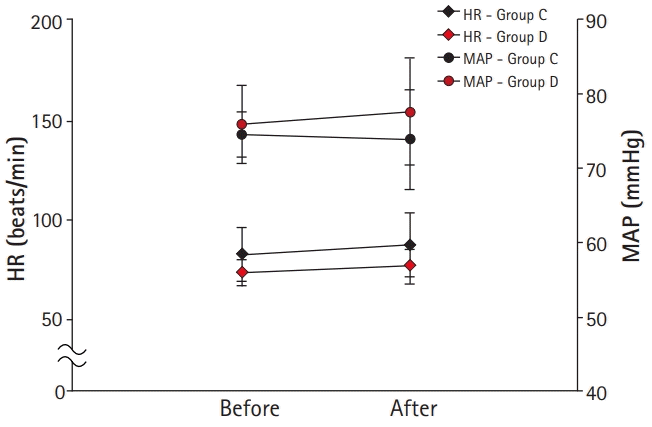
Fig. 4.
Transient hyperemic response test. F1: pre-compression systolic MCAFV, F2: systolic MCAFV during compression, F3: post compression systolic MCAFV, MCAFV; middle cerebral artery velocity.

Table 1.
Patient Demographics and Intraoperative Parameters
Table 2.
Comparison of Autoregulatory Tests