Abstract
Arginine deprivation is a novel antimetabolite strategy for the treatment of arginine-dependent cancers that exploits differential expression and regulation of key urea cycle enzymes. Several studies have focused on inactivation of argininosuccinate synthetase 1 (ASS1) in a range of malignancies, including melanoma, hepatocellular carcinoma (HCC), mesothelial and urological cancers, sarcomas, and lymphomas. Epigenetic silencing has been identified as a key mechanism for loss of the tumor suppressor role of ASS1 leading to tumoral dependence on exogenous arginine. More recently, dysregulation of argininosuccinate lyase has been documented in a subset of arginine auxotrophic glioblastoma multiforme, HCC and in fumarate hydratase-mutant renal cancers. Clinical trials of several arginine depletors are ongoing, including pegylated arginine deiminase (ADI-PEG20, Polaris Group) and bioengineered forms of human arginase. ADI-PEG20 is furthest along the path of clinical development from combinatorial phase 1 to phase 3 trials and is described in more detail. The challenge will be to identify tumors sensitive to drugs such as ADI-PEG20 and integrate these agents into multimodality drug regimens using imaging and tissue/fluid-based biomarkers as predictors of response. Lastly, resistance pathways to arginine deprivation require further study to optimize arginine-targeted therapies in the oncology clinic.
For many human cancers, dysregulation of cellular metabolism is now recognized as a key event in tumor growth and development. Accordingly, research into various aspects of tumor metabolism is undergoing a period of sustained revival, with the hope that this will identify novel tractable pathways for cancer screening, diagnosis and the treatment of malignant disease [1]. Indeed, the development of antimetabolites, such as antifolates and pyrimidine analogues, and the application of Warburg's hypothesis in 18F-fludeoxyglucose positron emission tomography/computed tomography (18F-FDG PET/CT) as a diagnostic and therapeutic imaging tool, demonstrate the importance of studying cancer metabolism in the oncology clinic: continued dissection of the cancer metabolome is likely to further refine our management of patients with cancer. Testing for metabolic genetic drivers such as mutations in Kreb's cycle genes (i.e., fumarate hydratase [FH], succinate dehydrogenase [SDH], isocitrate dehydrogenase-1/2 [IDH 1/2]) is already available, and new PET tracers and antimetabolites are being devised that aim to exploit the differential biochemistry of sugars, lipids and amino acids in normal and malignant cells [2,3]. Here, the focus is on the amino acid L-arginine, which has garnered clinical interest as an exciting cancer target, driven by the emergence of several novel arginine-depleting agents.
The rationale for targeting amino acids in cancer therapy was pioneered with the introduction of L-asparaginase in childhood leukemia over five decades ago [4]. In contrast to normal cells, leukemic cells are dependent on exogenous asparagine for growth (i.e., auxotrophic), due to lack of the biosynthetic enzyme asparagine synthetase [5]. As a monotherapy, deamidation of asparagine to aspartate and ammonia by L-asparaginase, led to responses of up to 50% in early studies of patients with chemorefractory leukemia [6]. However, it was not until the advent of multimodality chemotherapy regimens containing L-asparaginase, that the cure rate of acute lymphoblastic leukemia increased from 5% in the 1950's to the present 90%. The problem of hypersensitivity reactions and anti-drug neutralizing antibodies has been addressed to some extent by use of pegylation technology, switching to alternative L-asparaginase enzymes and individualized schedules with close monitoring of plasma asparagine levels [7]. Nevertheless, wider clinical investigation of L-asparaginase, has been hampered by the risk of severe dose-limiting side effects including hepatitis, pancreatitis, coagulopathy and neurotoxicity [8]. Bioengineering efforts are underway to modulate the secondary glutaminolytic activity that mediates, in part, the adverse effects but also the antitumor properties of L-asparaginase [9].
Based on the asparaginase paradigm, several argininecatabolizing enzymes have been developed, including mycoplasma-derived arginine deiminase and human recombinant arginase, for cancer therapy [10]. These enzymes are pegylated for enhanced pharmacokinetics and pharmacodynamics; however, further drug optimization will still be needed for arginine deprivation to gain traction in the clinic [11]. Although the data are preliminary, early signs suggest that arginine deprivation has the potential to make an impact across a broad range of human malignancies.
On an organismal level, diet and protein degradation are the main sources of plasma arginine, with only 5-15% (up to 30% in newborns) derived from de novo biosynthesis via the intestinal-renal axis. Indeed, under physiological conditions the arginine biosynthetic pathway is organized according to tissue function: for example, as the urea cycle in the liver for elimination of nitrogenous waste, and as the nitric oxide (NO)-citrulline cycle in endothelial cells to generate NO, with arginine acting as an intermediary molecule [12].
Numerous studies have confirmed that ASS1, considered a house-keeping gene in normal cells and a rate-limiting biosynthetic enzyme in hepatocytes and endothelial cells, is absent in many tumors [13,14]. Importantly, this leads to a critical dependence on exogenous arginine, with evidence suggesting that tumoral ASS1 deficiency may be both a prognostic biomarker and predictor of sensitivity to arginine deprivation therapy [15]. Methylation-dependent transcriptional silencing has been shown to drive tumoral ASS1 loss in some tumor types, although other mechanisms may also be involved, including repression of the ASS1 promoter by hypoxia-inducible factor-1alpha detected in melanoma cells [16,17]. Further study of the mechanisms of ASS1 loss and sensitivity to arginine depletion will be critical in overcoming resistance in the clinic.
The reason for down-regulation of tumoral ASS1 expression remains unclear and is an area of ongoing research. Recent studies have shown that ASS1 functions as a tumor suppressor in sarcoma and bladder cancer cell lines, reducing colony forming ability, proliferation and invasion of tumor cells and abrogating growth of tumor xenografts in mouse models [18-20]. Indeed, it appears that the reprogramming of tumor arginine metabolism by deactivating ASS1 favors a more aggressive phenotype fueled by exogenous arginine. Previously extracellular arginine has been shown to increase DNA synthesis in Burkitt lymphoma cells, and enhance incorporation of glutamine into nucleotides in colorectal cancer cells [21,22]. Work from our laboratory supports the hypothesis that exogenous arginine is sparing for glutamine in the synthesis of pyrimidines, specifically in ASS1-negative tumor cells. Thus, arginine depletion in ASS1-negative cells led to a marked increase in intracellular glutamine whilst thymidine levels declined secondary to inhibition of thymidylate synthase and dihydrofolate reductase, highlighting the interdependence between exogenous arginine and glutamine for nucleotide synthesis. In contrast, thymidine levels were unaffected by arginine withdrawal in ASS1-positive tumor cells, suggesting that glutamine is being diverted towards arginine synthesis, thereby limiting the capacity for proliferation and growth [20]. Evidence for crosstalk between glutamine and arginine has also been revealed in studies of melanoma cells exposed to ADI-PEG20 [23]. Moreover, metabolomic studies in sarcomas have identified arginine along with glutamine and serine, as essential amino acids with a signature of enhanced gemcitabine sensitivity in a subgroup of liposarcomas [24,25].
Notably, there is also evidence for an aberrant arginine metabolome in nasopharyngeal and bladder cancer cells with an inverse relationship between ASS1 and the expression of key serine pathway enzymes, including phosphoserine phosphatase (PSPH) and phosphoserine aminotransferase I (PSAT) [20,26]. Elevated levels of PSPH and PSAT have been identified in several tumors, and modulate one-carbon folate metabolism [27]. This is an area that will require detailed metabolomic studies to delineate the role of arginine given that there is increasing evidence for differential mechanisms involved in loss of ASS1 expression.
Less is known about the tumoral role of ASL, which is immediately downstream of ASS1 and catalyzes the conversion of argininosuccinate into arginine and fumarate. It is apparent from inborn errors of metabolism that ASL is critical in channeling arginine for NO production, with NO donors reversing the clinical picture of hypertension in children with ASL deficiency [28]. More recently, ASL has been explored in cancer, with upregulation noted in hepatocellular carcinoma (HCC) linked to increased aggressiveness mediated by NO and cyclin A2 signaling [29]. On the other hand, there is evidence that methylated ASL contributes to the arginine auxotrophy of glioblastoma multiforme, with loss of ASS1 and ASL conferring greater sensitivity to ADI-PEG20 [30]. In FH deficient renal cell cancer (RCC), where ASL is intact, the enzyme's activity is reversed producing high levels of argininosuccinate from arginine and fumarate. Here, arginosuccinate levels correlate with reversed ASL activity and may prove to be a biomarker in FH null RCC and other tumors [31,32]. Here too, the role of ASL in human cancer appears to be dependent on tumor type, and further work is needed to understand the implications of ASL expression in human cancer.
Lastly, tumoral heterogeneity of urea-cycle enzyme expression is likely to impact the development of arginine deprivation as a targeted therapy in human cancer. Concerning ASS1, whilst in some tumors, such as melanoma, lymphoma, and small cell lung cancer, there appears to be an 'all or none' level of expression, in the epithelial cancers, non-small cell lung carcinoma, mesothelioma and ovarian cancer, we have noted significant heterogeneity of expression (Fig. 1) [14,16,33-35]. As a result, moving forward into clinical trials we have opted for at least 50% ASS1 negativity within a tumor biopsy for patient selection, thus lowering the bar for patient inclusion, whereas previous trials in melanoma and small cell lung carcinoma (ClinicalTrials.gov Identifier: NCT01266018) have mandated at least 95% ASS1-negative cells.
Several decades of research have led to the notion that arginine promotes tumor growth: manipulation of dietary arginine in cancer cell line studies and in vivo transplantable tumor models; ex vivo analyses of cancer tissue arginase; and in vitro and in vivo pharmacological studies of native arginine-degrading enzymes [36-41]. More recent studies evaluating the efficacy of pegylated bioengineered enzymes have confirmed that arginine depletion is effective in various ASS1-deficient xenograft tumor models, including HCC, melanoma, pancreatic and small cell lung cancer and leukemia [34,42-44]. Whilst amino acid deprivation is known to induce nutritional starvation, the inhibitory effects of arginine depleting agents on tumor growth are not fully understood and remain an area of active investigation. In addition to the links between exogenous arginine and intracellular glutamine and pyrimidine synthesis described above-which impact directly on the cell cycle-ADI-PEG20 also downregulates mammalian target of rapamycin (mTOR) and modulates phosphoinositide 3-kinase (PI3K) via suppression of phosphatase and tensin homolog (PTEN) in ASS1-negative tumor cells [45-49]. It is noteworthy that arginine is one of two key amino acid regulators of mTOR-the other being leucine-and may explain, in part, the ability of arginine depletors to critically impair protein synthesis [50,51]. Apart from modulating tumor biomass via suppressing nucleotide and protein synthesis, arginine also affects tumor cell motility via a NO and focal adhesion kinase (FAK)-dependent pathway; importantly, FAK activation has been linked to deprivation of several other amino acids including glutamine and tyrosine [52-54]. Interestingly, arginine deiminase was identified in an anti-angiogenic screen and an anti-vascular effect has been confirmed both in HUVEC cells in vitro and in a xenograft melanoma study using fluorescence molecular tomography. Furthermore, arginine deiminase was antiangiogenic and potentiated the effect of radiation in a neuroblastoma mouse model [55-58]. Although some native arginine deiminases, found in micro-organisms such as Giardia lamblia, have functions beyond arginine depletion including citrullination of proteins (i.e., a peptidylarginine deiminase), this is not a feature of mycoplasmal-derived ADI-PEG20 used in clinical trials to date [59]. Clearly, arginine-lowering enzymes are likely to impact numerous other pathophysiological pathways and their further characterization will be crucial in optimizing arginine deprivation in the clinic [60-63].
Drug resistance remains a significant challenge for effective usage of arginine depletors. Despite promising results observed in vitro and in vivo as a targeted therapy for arginine auxotrophic tumors, several mechanisms have been identified that mediate tumoral resistance to arginine depletors. The most plausible is re-expression of ASS1, which results in the re-cycling of citrulline back into arginine [64]. This has been described for melanoma cells via transcriptional activation of the ASS1 promoter by the oncogene c-myc [17]. More recently, c-myc has been shown to play a role in the metabolic reprogramming seen during the development of ADI-PEG20 resistance in arginine auxotrophic melanoma cell lines. Here, ADI-PEG20-resistant cells exhibited elevated expression of key enzymes in glutaminolysis, glutaminase (GLS1) and glutamine dehydrogenase, and increased glycolytic metabolic activity, resulting in preferential sensitivity to glutamine inhibitors and glycolytic inhibitors, respectively. c-Myc, not elevated ASS1 expression, was found to contribute to this metabolic rewiring [17,23].
Autophagy ('self-eating'), leading to the provision of a temporary but finite supply of arginine via the breakdown of intracellular organelles, is also triggered by arginine deprivation, circumventing arginine deprivation in several malignancies, including prostate cancer, lymphoma, glioblastoma multiforme, and melanoma [16,30,65,66]. An alternative mechanism may involve macropinocytosis whereby extracellular proteins are degraded to supply the tumor cell with amino acids, as shown recently for K-ras transformed cells deprived of exogenous glutamine [67]. Finally, the direct supply of arginine or its precursors by the tumor microenvironment is also an attractive hypothesis. A similar hypothesis was first proposed and validated for mesenchymal-derived stromal cells providing asparagine for L-asparaginase-resistant acute lymphoblastic leukemia cells and, latterly, adipocytes supplying glutamine, consistent with the dual catalytic activity of L-asparaginase [68,69]. Evidence suggests that tumor associated macrophages (TAMs), predominant cells of the innate immune system within the tumor microenvironment, are involved in complex chemical cross-talk with tumor cells. TAMs are highly versatile and this interplay has the potential to modulate TAM phenotype, which we propose may in turn lead to a form of metabolic cooperation between TAMs and the tumor cells, resulting in the provision of arginine or its precursors to the tumor, thereby by-passing the effect of arginine deprivation. We have recently reviewed the complexity of the tumor microenvironment in relation to arginine, and it is clear that host cells may influence tumorigenesis by being sources of arginase as well as arginine [70-73]. Irrespective of the resistance mechanism, the final common pathway ends with arginine, as this is essential to the survival of the ASS1-negative tumor cell that is unable to supply sufficient amounts of arginine via upregulation of ASS1.
It is likely that further tumoral mechanisms involved in the efficacy and resistance of arginine depletors will be identified and this will be critical in exploiting arginine deprivation with rationally selected drugs for cancer therapy. Ultimately, this may improve the treatment efficacy of many cancers that depend on arginine for survival. To date several preclinical combinatorial studies have shown additive and/or synergistic effects of arginine depletors with taxanes, 5-fluorouracil, pemetrexed cytarabine, PI3K inhibitors, arginine mimetics (e.g., canavanine) and modulators of autophagy using chloroquine and TRAIL [20,44,45,74-77]. Additional interactions that merit further investigation include the potentiation observed between arginine deprivation and radiotherapy in 3D spheroid models of arginine auxotrophic cell lines [78]. Indeed, recent work indicates that ADI-PEG20 is synergistic in vitro and in vivo with radiotherapy in pancreatic cancer cells and may have utility in combination with gemcitabine, a known radiosensitizer and potentiator of ADI-PEG20 in pancreatic malignancy [79,80].
We have investigated further the effect of combining ADI-PEG20 with the antifolate drug pemetrexed based on the novel finding that ADI-PEG20 suppresses the pemetrexed target enzymes, thymidylate synthase (TS) and dihydrofolate reductase (DHFR). It is noteworthy that high TS expression, in particular, has been linked to poor clinical outcome and resistance to antifolates in several cancers including the bladder, lung, mesothelium, and colon [81-85]. Moreover, we showed that loss of ASS1 was correlated with high levels of TS and DHFR and predicted for a poor outcome in patients with transitional carcinoma of the bladder. Subsequently, we validated that arginine deprivation with ADI-PEG20 potentiated the effect of pemetrexed with enhanced apoptosis as assessed by cleaved poly (ADP-ribose) polymerase and annexin V staining. Furthermore, decreased 18F-fluorothymidine (FLT) uptake by PET may be utilized as a predictive imaging biomarker in selecting tumors susceptible to this drug combination (see below).
Specific mention should be made here regarding platinum compounds and their relationship to ASS1 and arginine auxotrophy. Helleman et al. [86] first identified loss of ASS1 as one of 9 genes that predicted independently for cisplatin resistance in a microarray retrospective study of patients with ovarian cancer [87]. Subsequently ASS1 gene overexpression and knock-down in ovarian cancer cells confirmed that while ASS1 loss conferred platinum resistance, there was collateral sensitivity to arginine deprivation [88]. In contrast, studies linking ASS1 loss to enhanced platinum salt sensitivity, namely oxaliplatin and human arginase in HCC cell lines, and cisplatin and ADI-PEG20 in melanoma cells, may exploit a prosurvival pathway that depends on NO synthesis derived from exogenous arginine [89,90]. Consistent with this hypothesis, targeted inhibition of inducible NO synthase synergizes with cisplatin chemotherapy in the treatment of arginine-auxotrophic melanoma cells [91,92].
Lastly, there is increasing interest in the development of novel types of human arginase, in particular a pegylated cobalt-modified (i.e., substituted for native manganese) enzyme (HuArg I [Co]-PEG5K) that has shown good efficacy in depleting arginine [93,94]. Animal toxicity testing indicates that citrulline supplementation is required since arginase, unlike ADI, produces ornithine which cannot be recycled readily to citrulline [95]. Several studies confirm that HuArg I [Co]-PEG5K displays tumor cytotoxicity in vitro and is active in xenograft tumor models [96,97].
The initial phase I/II studies of ADI-PEG20 were performed in patients with liver cancer and melanoma, tumors with a high frequency of ASS1 loss (Table 1) [33,98-105]. Following the first intramuscular injection of 160 IU/m2 of ADI-PEG20, plasma arginine levels decrease by 24 hours and remain low for at least seven days. ADI-PEG20 therapy also triggers a reciprocal increase in plasma citrulline and a decline in plasma nitrite and nitrate levels due to reduced NO synthesis. Response rates of between 25-47% were observed in these small studies with good safety and tolerability [98,99]. The common adverse reactions have been self-limiting grade 1-2 injection site reactions, less frequently diffuse skin rashes and arthralgia, and rarely neutropenia, anaphylactoid reactions and serum sickness. The latest ADI-PEG20 clinical trials have recorded stable disease as the best response, with evidence for a rebound in arginine plasma levels due to drug neutralising antibodies that limit the duration of arginine depletion to a median of 50 days [100,101]. However, as pharmacodymanic measurements were made eight days apart it is possible that more frequent blood testing may have detected oscillations in plasma arginine and this needs further study. Notably, Yang et al. [102] performed a randomized phase 2 study of two different doses of ADI-PEG20 (320 IU/m2 vs. 160 IU/m2) in HCC showing that disease control rates were similar in both groups. A trend towards better survival was observed in patients with >4 weeks (10.0 months) compared with <4 weeks (5.8 months) of arginine deprivation. Based on these encouraging results, a large phase 3 trial (ClinicalTrials.gov Identifier: NCT01 287585) is currently accruing in HCC using the 160 IU/m2 dose with results expected in 2015.
The role of ASS1 as a predictor of response to ADI-PEG20 has been explored only recently in clinical trials. Feun et al. [33] assessed the role of ASS1 expression in a trial of ADI-PEG20 in patients with metastatic melanoma. Responses were confined to patients with ASS1-negative tumors, and were seen only with the higher ADI-PEG20 dose of 320 IU/m2. An improvement in the progression-free survival (PFS) was detected in patients with ASS1-negative compared to ASS1-positive tumors (p=0.025) however no overall survival (OS) difference was observed in this small study. In turn, we have conducted the first prospective multicenter randomized study of ADI-PEG20 in patients pre-selected for ASS1 deficiency in cancer by focusing on the asbestos-related cancer mesothelioma (arginine deiminase and mesothelioma or ADAM). ADAM met its primary endpoint of an improvement in the PFS in the best supportive care (BSC) plus ADI-PEG20 group compared to the BSC alone group of almost 6 weeks (p=0.02) [103]. Our data support differential methylation as the basis for the loss of ASS1 observed in mesothelioma, consistent with our earlier cell line studies.
Thus far, human trials of arginase have been confined to a small phase I study in patients with HCC using peg-rhArgI (BCT, Hong Kong, China), a human pegylated enzyme derived from an Escherichia coli expression system [104]. In contrast to ADI-PEG20, peg-rhArgI was given intravenously (I.V.) and reduced plasma arginine to undetectable levels throughout the treatment period with no evidence for neutralizing antibodies. Interestingly, the PFS and OS for the peg-rhArgI are consistent with that observed in trials of ADI-PEG20 in heavily pre-treated patients with HCC and suggest that the ability to switch on tumoral resistance mechanisms may be critical in determining efficacy to arginine depletors, rather than the duration of arginine depletion per se.
Several groups have assessed potential imaging biomarkers of tumor response to arginine deprivation. Ott et al. [101] assessed the effect of arginine deprivation with ADI-PEG20 on metastatic melanoma with both early and late imaging timepoints "using PET." Partial metabolic responses (PMRs) were detected at the early time point in ~25% of patients (day 4), but were not sustained by the late time point (56 days), likely due to a combination of early resistance (e.g., re-expression of ASS1 and induction of autophagy) and a rebound in plasma arginine levels. We also performed early time point imaging (day 15) in the ADAM trial, and while by modified Response Evaluation Criteria in Solid Tumors (RECIST) we confirmed stable disease as the best response, almost 50% of patients had evidence of a PMR by imaging with 18F-FDG PET/CT [103,105].
One of the drawbacks in imaging some arginine auxotrophs such as melanoma, is the apparent increase in glucose uptake following ADI-PEG20 therapy secondary to PTEN loss and PI3K-induced glucose transporter type 1 (GLUT-1) expression [47]. Thus, we and others have explored FLT as an alternative imaging PET tracer and although this does not appear predictive in ASS1-negative melanoma, our studies confirmed robust suppression of FLT uptake following ADI-PEG20 treatment of ASS1-methylated tumors [20,106]. Moreover, the uptake of FLT correlated directly with thymidine-kinase 1 (TK1) protein levels consistent with the mode of action of ADI-PEG20 in modulating protein turnover. A trial is planned to explore the role of FLT in patients with ASS1-deficient thoracic cancers as part of a phase I/II study discussed in more detail below.
Targeting arginine is moving towards combinatorial studies based on the emerging preclinical data of additive and synergistic drug interactions in the treatment of arginine auxotrophic cancers. Several ADI-PEG20 combination trials are ongoing or planned and follow the developmental template for asparaginase several decades ago (summarized in Table 2). Studies of the doublet of ADI-PEG20 and cisplatin and ADI-PEG20 and docetaxel to date have shown no untoward toxicity other than that ascribed to either agent alone (Bomalaski J, personal communication).
Building on the ADAM trial, we have hypothesized that ADI-PEG20 with the standard doublet of cisplatin and pemetrexed may provide a novel way of targeting the heterogeneity and chemoresistance of mesothelioma. As previously mentioned, several epithelial tumors, including mesothelioma and non-small cell lung cancer, harbor cells with varying degrees of dependence on exogenous arginine as inferred by the differential expression of ASS1. Since ASS1 positivity is a biomarker of cisplatin sensitivity whereas ASS1 loss is a predictor of cisplatin resistance but collateral sensitivity to ADI-PEG20-pemetrexed, the triplet regimen may be superior to standard chemotherapy alone (Fig. 2). Once the maximum tolerated dose has been defined from the phase 1 triplet combination study, early FLT-PET/CT will be assessed as a potential biomarker of response in patients with thoracic malignancy. Patients will be imaged at baseline and at 24hrs following a single dose of ADI-PEG20 with additional FLTPET/CT scans after the first cycle of chemotherapy and upon completion of all treatment.
From the preclinical studies referred to above, the role of combining arginine deprivation with autophagy inhibitors, glutamine and glycolytic inhibitors, modulators of the tumor microenvironment and radiotherapy, are just a few of the exciting questions to explore in the context of future clinical trials.
Arginine deprivation has the potential to improve the management of several hard-to-treat cancers by exploiting differential expression and regulation of key urea cycle enzymes, ASS1 and ASL. Clinical trials that employ biomarkers for patient selection and treatment response are needed to optimize arginine deprivation therapy in cancer. The field is evolving towards combination strategies and it is hoped that arginine-targeted therapies will soon become part of the standard cancer armamentarium.
References
1. Hanahan D, Weinberg RA. Hallmarks of cancer: the next generation. Cell. 2011; 144:646–674. PMID: 21376230.


2. Schiffman JD. No child left behind in SDHB testing for paragangliomas and pheochromocytomas. J Clin Oncol. 2011; 29:4070–4072. PMID: 21969491.


3. Vander Heiden MG. Targeting cancer metabolism: a therapeutic window opens. Nat Rev Drug Discov. 2011; 10:671–684. PMID: 21878982.


4. Broome JD. Evidence that the L-asparaginase activity of Guinea pig serum is responsible for its antilymphoma effects. Nature. 1961; 191:1114–1115.


5. Richards NG, Kilberg MS. Asparagine synthetase chemotherapy. Annu Rev Biochem. 2006; 75:629–654. PMID: 16756505.


6. Jaffe N, Traggis D, Das L, Frauenberger G, Hann HW, Kim BS, et al. Favorable remission induction rate with twice weekly doses of L-asparaginase. Cancer Res. 1973; 33:1–4. PMID: 4565907.
7. Douer D. Is asparaginase a critical component in the treatment of acute lymphoblastic leukemia? Best Pract Res Clin Haematol. 2008; 21:647–658. PMID: 19041604.


8. Truelove E, Fielding AK, Hunt BJ. The coagulopathy and thrombotic risk associated with L-asparaginase treatment in adults with acute lymphoblastic leukaemia. Leukemia. 2013; 27:553–559. PMID: 23099335.


9. Offman MN, Krol M, Patel N, Krishnan S, Liu J, Saha V, et al. Rational engineering of L-asparaginase reveals importance of dual activity for cancer cell toxicity. Blood. 2011; 117:1614–1621. PMID: 21106986.


10. Wheatley DN. Controlling cancer by restricting arginine availability: arginine-catabolizing enzymes as anticancer agents. Anticancer Drugs. 2004; 15:825–833. PMID: 15457122.
11. Feun L, Savaraj N. Pegylated arginine deiminase: a novel anticancer enzyme agent. Expert Opin Investig Drugs. 2006; 15:815–822.


12. Husson A, Brasse-Lagnel C, Fairand A, Renouf S, Lavoinne A. Argininosuccinate synthetase from the urea cycle to the citrulline-NO cycle. Eur J Biochem. 2003; 270:1887–1899. PMID: 12709047.


13. Dillon BJ, Prieto VG, Curley SA, Ensor CM, Holtsberg FW, Bomalaski JS, et al. Incidence and distribution of argininosuccinate synthetase deficiency in human cancers: a method for identifying cancers sensitive to arginine deprivation. Cancer. 2004; 100:826–833. PMID: 14770441.
14. Szlosarek PW, Grimshaw MJ, Wilbanks GD, Hagemann T, Wilson JL, Burke F, et al. Aberrant regulation of argininosuccinate synthetase by TNF-alpha in human epithelial ovarian cancer. Int J Cancer. 2007; 121:6–11. PMID: 17354225.
15. Delage B, Fennell DA, Nicholson L, McNeish I, Lemoine NR, Crook T, et al. Arginine deprivation and argininosuccinate synthetase expression in the treatment of cancer. Int J Cancer. 2010; 126:2762–2772. PMID: 20104527.


16. Delage B, Luong P, Maharaj L, O'Riain C, Syed N, Crook T, et al. Promoter methylation of argininosuccinate synthetase-1 sensitises lymphomas to arginine deiminase treatment, autophagy and caspase-dependent apoptosis. Cell Death Dis. 2012; 3:e342. PMID: 22764101.


17. Tsai WB, Aiba I, Lee SY, Feun L, Savaraj N, Kuo MT. Resistance to arginine deiminase treatment in melanoma cells is associated with induced argininosuccinate synthetase expression involving c-Myc/HIF-1alpha/Sp4. Mol Cancer Ther. 2009; 8:3223–3233. PMID: 19934275.
18. Kobayashi E, Masuda M, Nakayama R, Ichikawa H, Satow R, Shitashige M, et al. Reduced argininosuccinate synthetase is a predictive biomarker for the development of pulmonary metastasis in patients with osteosarcoma. Mol Cancer Ther. 2010; 9:535–544. PMID: 20159990.


19. Huang HY, Wu WR, Wang YH, Wang JW, Fang FM, Tsai JW, et al. ASS1 as a novel tumor suppressor gene in myxofibrosarcomas: aberrant loss via epigenetic DNA methylation confers aggressive phenotypes, negative prognostic impact, and therapeutic relevance. Clin Cancer Res. 2013; 19:2861–2872. PMID: 23549872.


20. Allen M, Luong P, Hudson C, Leyton J, Delage B, Ghazaly E, et al. Prognostic and therapeutic impact of argininosuccinate synthetase-1 control in bladder cancer as monitored longitudinally by PET imaging. Cancer Res. 2013; 11. 27. [Epub]. http://dx.doi.org/10.1158/0008-5472.CAN-13-1702
.


21. Osunkoya BO, Adler WH, Smith RT. Effect of arginine deficiency on synthesis of DNA and immunoglobulin receptor of Burkitt lymphoma cells. Nature. 1970; 227:398–399. PMID: 4193643.


22. Yamauchi K, Komatsu T, Kulkarni AD, Ohmori Y, Minami H, Ushiyama Y, et al. Glutamine and arginine affect Caco-2 cell proliferation by promotion of nucleotide synthesis. Nutrition. 2002; 18:329–333. PMID: 11934546.


23. Long Y, Tsai WB, Wangpaichitr M, Tsukamoto T, Savaraj N, Feun LG, et al. Arginine deiminase resistance in melanoma cells is associated with metabolic reprogramming, glucose dependence, and glutamine addiction. Mol Cancer Ther. 2013; 12:2581–2590. PMID: 23979920.


24. Braas D, Ahler E, Tam B, Nathanson D, Riedinger M, Benz MR, et al. Metabolomics strategy reveals subpopulation of liposarcomas sensitive to gemcitabine treatment. Cancer Discov. 2012; 2:1109–1117. PMID: 23230188.


25. Van Tine BA, Bean GR, Boone P, Tanas M, Schulze MB, Chen DY, et al. Using pegylated arginine deiminase (ADI-PEG20) for the treatment of sarcomas that lack argininosuccinate synthesase 1 expression. J Clin Oncol. 2013; 31(S):10526.


26. Lan J, Tai HC, Lee SW, Chen TJ, Huang HY, Li CF. Deficiency in expression and epigenetic DNA methylation of ASS1 gene in nasopharyngeal carcinoma: negative prognostic impact and therapeutic relevance. Tumour Biol. 2013; 7. 30. [Epub]. http://dx.doi.org/10.1007/s13277-013-1020-8
.


27. Possemato R, Marks KM, Shaul YD, Pacold ME, Kim D, Birsoy K, et al. Functional genomics reveal that the serine synthesis pathway is essential in breast cancer. Nature. 2011; 476:346–350. PMID: 21760589.


28. Erez A, Nagamani SC, Shchelochkov OA, Premkumar MH, Campeau PM, Chen Y, et al. Requirement of argininosuccinate lyase for systemic nitric oxide production. Nat Med. 2011; 17:1619–1626. PMID: 22081021.


29. Huang HL, Hsu HP, Shieh SC, Chang YS, Chen WC, Cho CY, et al. Attenuation of argininosuccinate lyase inhibits cancer growth via cyclin A2 and nitric oxide. Mol Cancer Ther. 2013; 12:2505–2516. PMID: 23979921.


30. Syed N, Langer J, Janczar K, Singh P, Lo Nigro C, Lattanzio L, et al. Epigenetic status of argininosuccinate synthetase and argininosuccinate lyase modulates autophagy and cell death in glioblastoma. Cell Death Dis. 2013; 4:e458. PMID: 23328665.


31. Zheng L, MacKenzie ED, Karim SA, Hedley A, Blyth K, Kalna G, et al. Reversed argininosuccinate lyase activity in fumarate hydratase-deficient cancer cells. Cancer Metab. 2013; 1:12. PMID: 24280230.


32. Adam J, Yang M, Bauerschmidt C, Kitagawa M, O'Flaherty L, Maheswaran P, et al. A role for cytosolic fumarate hydratase in urea cycle metabolism and renal neoplasia. Cell Rep. 2013; 3:1440–1448. PMID: 23643539.


33. Feun LG, Marini A, Walker G, Elgart G, Moffat F, Rodgers SE, et al. Negative argininosuccinate synthetase expression in melanoma tumours may predict clinical benefit from arginine-depleting therapy with pegylated arginine deiminase. Br J Cancer. 2012; 106:1481–1485. PMID: 22472884.


34. Kelly MP, Jungbluth AA, Wu BW, Bomalaski J, Old LJ, Ritter G. Arginine deiminase PEG20 inhibits growth of small cell lung cancers lacking expression of argininosuccinate synthetase. Br J Cancer. 2012; 106:324–332. PMID: 22134507.


35. Szlosarek PW, Klabatsa A, Pallaska A, Sheaff M, Smith P, Crook T, et al. In vivo loss of expression of argininosuccinate synthetase in malignant pleural mesothelioma is a biomarker for susceptibility to arginine depletion. Clin Cancer Res. 2006; 12:7126–7131. PMID: 17145837.


36. Gilroy E. The influence of arginine upon the growth rate of a transplantable tumour in the mouse. Biochem J. 1930; 24:589–595. PMID: 16744397.


37. Yeatman TJ, Risley GL, Brunson ME. Depletion of dietary arginine inhibits growth of metastatic tumor. Arch Surg. 1991; 126:1376–1381. PMID: 1747050.


38. Bach SJ, Lasnitzki I. Some aspects of the role of arginine and arginase in mouse carcinoma 63. Enzymologia. 1947; 12:198–205. PMID: 18910560.
39. Storr JM, Burton AF. The effects of arginine deficiency on lymphoma cells. Br J Cancer. 1974; 30:50–59. PMID: 4528778.


40. Scott L, Lamb J, Smith S, Wheatley DN. Single amino acid (arginine) deprivation: rapid and selective death of cultured transformed and malignant cells. Br J Cancer. 2000; 83:800–810. PMID: 10952786.


41. Takaku H, Takase M, Abe S, Hayashi H, Miyazaki K. In vivo anti-tumor activity of arginine deiminase purified from Mycoplasma arginini. Int J Cancer. 1992; 51:244–249. PMID: 1568792.
42. Ensor CM, Holtsberg FW, Bomalaski JS, Clark MA. Pegylated arginine deiminase (ADI-SS PEG20,000 mw) inhibits human melanomas and hepatocellular carcinomas in vitro and in vivo. Cancer Res. 2002; 62:5443–5450. PMID: 12359751.
43. Bowles TL, Kim R, Galante J, Parsons CM, Virudachalam S, Kung HJ, et al. Pancreatic cancer cell lines deficient in argininosuccinate synthetase are sensitive to arginine deprivation by arginine deiminase. Int J Cancer. 2008; 123:1950–1955. PMID: 18661517.


44. Hernandez CP, Morrow K, Lopez-Barcons LA, Zabaleta J, Sierra R, Velasco C, et al. Pegylated arginase I: a potential therapeutic approach in T-ALL. Blood. 2010; 115:5214–5221. PMID: 20407034.


45. Kim RH, Coates JM, Bowles TL, McNerney GP, Sutcliffe J, Jung JU, et al. Arginine deiminase as a novel therapy for prostate cancer induces autophagy and caspase-independent apoptosis. Cancer Res. 2009; 69:700–708. PMID: 19147587.


46. Wu FL, Liang YF, Chang YC, Yo HH, Wei MF, Shen LJ. RNA interference of argininosuccinate synthetase restores sensitivity to recombinant arginine deiminase (rADI) in resistant cancer cells. J Biomed Sci. 2011; 18:25. PMID: 21453546.


47. Stelter L, Evans MJ, Jungbluth AA, Zanzonico P, Ritter G, Ku T, et al. Novel mechanistic insights into arginine deiminase pharmacology suggest 18F-FDG is not suitable to evaluate clinical response in melanoma. J Nucl Med. 2012; 53:281–286. PMID: 22228793.


48. Gong H, Zolzer F, von Recklinghausen G, Rossler J, Breit S, Havers W, et al. Arginine deiminase inhibits cell proliferation by arresting cell cycle and inducing apoptosis. Biochem Biophys Res Commun. 1999; 261:10–14. PMID: 10405315.


49. Gong H, Zolzer F, von Recklinghausen G, Havers W, Schweigerer L. Arginine deiminase inhibits proliferation of human leukemia cells more potently than asparaginase by inducing cell cycle arrest and apoptosis. Leukemia. 2000; 14:826–829. PMID: 10803513.


50. Morrow K, Hernandez CP, Raber P, Del Valle L, Wilk AM, Majumdar S, et al. Anti-leukemic mechanisms of pegylated arginase I in acute lymphoblastic T-cell leukemia. Leukemia. 2013; 27:569–577. PMID: 22926702.


51. Laplante M, Sabatini DM. mTOR signaling in growth control and disease. Cell. 2012; 149:274–293. PMID: 22500797.


52. Rhoads JM, Chen W, Gookin J, Wu GY, Fu Q, Blikslager AT, et al. Arginine stimulates intestinal cell migration through a focal adhesion kinase dependent mechanism. Gut. 2004; 53:514–522. PMID: 15016745.


53. Rhoads JM, Liu Y, Niu X, Surendran S, Wu G. Arginine stimulates cdx2-transformed intestinal epithelial cell migration via a mechanism requiring both nitric oxide and phosphorylation of p70 S6 kinase. J Nutr. 2008; 138:1652–1657. PMID: 18716165.


54. Fu YM, Zhang H, Ding M, Li YQ, Fu X, Yu ZX, et al. Specific amino acid restriction inhibits attachment and spreading of human melanoma via modulation of the integrin/focal adhesion kinase pathway and actin cytoskeleton remodeling. Clin Exp Metastasis. 2004; 21:587–598. PMID: 15787096.


55. Wilm M, Shevchenko A, Houthaeve T, Breit S, Schweigerer L, Fotsis T, et al. Femtomole sequencing of proteins from polyacrylamide gels by nano-electrospray mass spectrometry. Nature. 1996; 379:466–469. PMID: 8559255.


56. Park IS, Kang SW, Shin YJ, Chae KY, Park MO, Kim MY, et al. Arginine deiminase: a potential inhibitor of angiogenesis and tumour growth. Br J Cancer. 2003; 89:907–914. PMID: 12942125.


57. Stelter L, Evans MJ, Jungbluth AA, Longo VA, Zanzonico P, Ritter G, et al. Imaging of tumor vascularization using fluorescence molecular tomography to monitor arginine deiminase treatment in melanoma. Mol Imaging. 2013; 12:67–73. PMID: 23348793.
58. Gong H, Pottgen C, Stuben G, Havers W, Stuschke M, Schweigerer L. Arginine deiminase and other antiangiogenic agents inhibit unfavorable neuroblastoma growth: potentiation by irradiation. Int J Cancer. 2003; 106:723–728. PMID: 12866032.


59. Touz MC, Ropolo AS, Rivero MR, Vranych CV, Conrad JT, Svard SG, et al. Arginine deiminase has multiple regulatory roles in the biology of Giardia lamblia. J Cell Sci. 2008; 121(Pt 17):2930–2938. PMID: 18697833.


60. Morris SM Jr. Recent advances in arginine metabolism: roles and regulation of the arginases. Br J Pharmacol. 2009; 157:922–930. PMID: 19508396.
61. Wu G, Morris SM Jr. Arginine metabolism: nitric oxide and beyond. Biochem J. 1998; 336(Pt 1):1–17. PMID: 9806879.


62. Leong HX, Simkevich C, Lesieur-Brooks A, Lau BW, Fugere C, Sabo E, et al. Short-term arginine deprivation results in large-scale modulation of hepatic gene expression in both normal and tumor cells: microarray bioinformatic analysis. Nutr Metab (Lond). 2006; 3:37. PMID: 16961918.


63. Brasse-Lagnel CG, Lavoinne AM, Husson AS. Amino acid regulation of mammalian gene expression in the intestine. Biochimie. 2010; 92:729–735. PMID: 20188788.


64. Shen LJ, Lin WC, Beloussow K, Shen WC. Resistance to the anti-proliferative activity of recombinant arginine deiminase in cell culture correlates with the endogenous enzyme, argininosuccinate synthetase. Cancer Lett. 2003; 191:165–170. PMID: 12618329.


65. Kim RH, Bold RJ, Kung HJ. ADI, autophagy and apoptosis: metabolic stress as a therapeutic option for prostate cancer. Autophagy. 2009; 5:567–568. PMID: 19276647.


66. Wang Z, Shi X, Li Y, Zeng X, Fan J, Sun Y, et al. Involvement of autophagy in recombinant human arginase-induced cell apoptosis and growth inhibition of malignant melanoma cells. Appl Microbiol Biotechnol. 2013; 8. 06. [Epub]. http://dx.doi.org/10.1007/s00253-013-5118-0
.


67. Commisso C, Davidson SM, Soydaner-Azeloglu RG, Parker SJ, Kamphorst JJ, Hackett S, et al. Macropinocytosis of protein is an amino acid supply route in Ras-transformed cells. Nature. 2013; 497:633–637. PMID: 23665962.


68. Iwamoto S, Mihara K, Downing JR, Pui CH, Campana D. Mesenchymal cells regulate the response of acute lymphoblastic leukemia cells to asparaginase. J Clin Invest. 2007; 117:1049–1057. PMID: 17380207.


69. Ehsanipour EA, Sheng X, Behan JW, Wang X, Butturini A, Avramis VI, et al. Adipocytes cause leukemia cell resistance to L-asparaginase via release of glutamine. Cancer Res. 2013; 73:2998–3006. PMID: 23585457.


70. Ellyard JI, Quah BJ, Simson L, Parish CR. Alternatively activated macrophage possess antitumor cytotoxicity that is induced by IL-4 and mediated by arginase-1. J Immunother. 2010; 33:443–452. PMID: 20463604.


71. Zea AH, Rodriguez PC, Atkins MB, Hernandez C, Signoretti S, Zabaleta J, et al. Arginase-producing myeloid suppressor cells in renal cell carcinoma patients: a mechanism of tumor evasion. Cancer Res. 2005; 65:3044–3048. PMID: 15833831.


72. Kwong-Lam F, Chi-Fung CG. Vincristine could partly suppress stromal support to T-ALL blasts during pegylated arginase I treatment. Exp Hematol Oncol. 2013; 2:11. PMID: 23574711.


73. Phillips M, Szlosarek PW. Arginine metabolism and tumour-associated macrophages. In : Lawrence T, Hagemann T, editors. Tumour-associated macrophages. New York: Springer;2012. p. 77–90.
74. Cheng PN, Lam TL, Lam WM, Tsui SM, Cheng AW, Lo WH, et al. Pegylated recombinant human arginase (rhArgpeg5,000mw) inhibits the in vitro and in vivo proliferation of human hepatocellular carcinoma through arginine depletion. Cancer Res. 2007; 67:309–317. PMID: 17210712.
75. Tsai WB, Aiba I, Long Y, Lin HK, Feun L, Savaraj N, et al. Activation of Ras/PI3K/ERK pathway induces c-Myc stabilization to upregulate argininosuccinate synthetase, leading to arginine deiminase resistance in melanoma cells. Cancer Res. 2012; 72:2622–2633. PMID: 22461507.


76. You M, Savaraj N, Kuo MT, Wangpaichitr M, Varona-Santos J, Wu C, et al. TRAIL induces autophagic protein cleavage through caspase activation in melanoma cell lines under arginine deprivation. Mol Cell Biochem. 2013; 374:181–190. PMID: 23180246.


77. Vynnytska BO, Mayevska OM, Kurlishchuk YV, Bobak YP, Stasyk OV. Canavanine augments proapoptotic effects of arginine deprivation in cultured human cancer cells. Anticancer Drugs. 2011; 22:148–157. PMID: 20717004.


78. Vynnytska-Myronovska B, Bobak Y, Garbe Y, Dittfeld C, Stasyk O, Kunz-Schughart LA. Single amino acid arginine starvation efficiently sensitizes cancer cells to canavanine treatment and irradiation. Int J Cancer. 2012; 130:2164–2175. PMID: 21647872.


80. Deorukhkar A, Diep N, Chatterjee D, Diagardjane P, Bomalaski J, Krishnan S. Arginine deiminase: a novel radiosensitizer in pancreatic cancer in vitro and in vivo. In : 2014 ASCO Gastrointestinal Cancers Symposium; 2014 Jan 16-18; San Francisco, CA. Abstr no. 221.
80. Daylami R, Muilenburg D, Bowles TL, Martinez SR, Bold RJ. Arginine deprivation by PEG-ADI induces autophagic cell death and enhances the tumor suppression effect of gemcitabine in pancreatic cancer. In : 2010 AACR 101st Annual Meeting; 2010 April 17-21; Washington, DC. Abstr no. 484.
81. Li Y, Li X, Dai H, Sun X, Li J, Yang F, et al. Thymidylate synthase was associated with patient prognosis and the response to adjuvant therapy in bladder cancer. BJU Int. 2009; 103:547–552. PMID: 18990150.


82. Takezawa K, Okamoto I, Okamoto W, Takeda M, Sakai K, Tsukioka S, et al. Thymidylate synthase as a determinant of pemetrexed sensitivity in non-small cell lung cancer. Br J Cancer. 2011; 104:1594–1601. PMID: 21487406.


83. Righi L, Papotti MG, Ceppi P, Bille A, Bacillo E, Molinaro L, et al. Thymidylate synthase but not excision repair crosscomplementation group 1 tumor expression predicts outcome in patients with malignant pleural mesothelioma treated with pemetrexed-based chemotherapy. J Clin Oncol. 2010; 28:1534–1539. PMID: 20177021.


84. Sigmond J, Backus HH, Wouters D, Temmink OH, Jansen G, Peters GJ. Induction of resistance to the multitargeted antifolate Pemetrexed (ALIMTA) in WiDr human colon cancer cells is associated with thymidylate synthase overexpression. Biochem Pharmacol. 2003; 66:431–438. PMID: 12907242.


85. Edler D, Kressner U, Ragnhammar P, Johnston PG, Magnusson I, Glimelius B, et al. Immunohistochemically detected thymidylate synthase in colorectal cancer: an independent prognostic factor of survival. Clin Cancer Res. 2000; 6:488–492. PMID: 10690528.
86. Helleman J, Jansen MP, Span PN, van Staveren IL, Massuger LF, Meijer-van Gelder ME, et al. Molecular profiling of platinum resistant ovarian cancer. Int J Cancer. 2006; 118:1963–1971. PMID: 16287073.


87. Melaiu O, Cristaudo A, Melissari E, Di Russo M, Bonotti A, Bruno R, et al. A review of transcriptome studies combined with data mining reveals novel potential markers of malignant pleural mesothelioma. Mutat Res. 2012; 750:132–140. PMID: 22198210.


88. Nicholson LJ, Smith PR, Hiller L, Szlosarek PW, Kimberley C, Sehouli J, et al. Epigenetic silencing of argininosuccinate synthetase confers resistance to platinum-induced cell death but collateral sensitivity to arginine auxotrophy in ovarian cancer. Int J Cancer. 2009; 125:1454–1463. PMID: 19533750.


89. Chow AK, Ng L, Sing Li H, Cheng CW, Lam CS, Yau TC, et al. Anti-tumor efficacy of a recombinant human arginase in human hepatocellular carcinoma. Curr Cancer Drug Targets. 2012; 12:1233–1243. PMID: 22873218.
90. Savaraj N, You M, Wu C, Wangpaichitr M, Kuo MT, Feun LG. Arginine deprivation, autophagy, apoptosis (AAA) for the treatment of melanoma. Curr Mol Med. 2010; 10:405–412. PMID: 20459375.


91. Sikora AG, Gelbard A, Davies MA, Sano D, Ekmekcioglu S, Kwon J, et al. Targeted inhibition of inducible nitric oxide synthase inhibits growth of human melanoma in vivo and synergizes with chemotherapy. Clin Cancer Res. 2010; 16:1834–1844. PMID: 20215556.


92. Grimm EA, Sikora AG, Ekmekcioglu S. Molecular pathways: inflammation-associated nitric-oxide production as a cancersupporting redox mechanism and a potential therapeutic target. Clin Cancer Res. 2013; 19:5557–5563. PMID: 23868870.


93. Stone E, Chantranupong L, Gonzalez C, O'Neal J, Rani M, VanDenBerg C, et al. Strategies for optimizing the serum persistence of engineered human arginase I for cancer therapy. J Control Release. 2012; 158:171–179. PMID: 22001609.


94. Agrawal V, Woo JH, Mauldin JP, Jo C, Stone EM, Georgiou G, et al. Cytotoxicity of human recombinant arginase I (Co)-PEG5000 in the presence of supplemental L-citrulline is dependent on decreased argininosuccinate synthetase expression in human cells. Anticancer Drugs. 2012; 23:51–64. PMID: 21955999.


95. Mauldin JP, Zeinali I, Kleypas K, Woo JH, Blackwood RS, Jo CH, et al. Recombinant human arginase toxicity in mice is reduced by citrulline supplementation. Transl Oncol. 2012; 5:26–31. PMID: 22348173.


96. Glazer ES, Stone EM, Zhu C, Massey KL, Hamir AN, Curley SA. Bioengineered human arginase I with enhanced activity and stability controls hepatocellular and pancreatic carcinoma xenografts. Transl Oncol. 2011; 4:138–146. PMID: 21633669.


97. Tanios R, Bekdash A, Kassab E, Stone E, Georgiou G, Frankel AE, et al. Human recombinant arginase I(Co)-PEG5000 [HuArgI(Co)-PEG5000]-induced arginine depletion is selectively cytotoxic to human acute myeloid leukemia cells. Leuk Res. 2013; 37:1565–1571. PMID: 24018014.


98. Izzo F, Marra P, Beneduce G, Castello G, Vallone P, De Rosa V, et al. Pegylated arginine deiminase treatment of patients with unresectable hepatocellular carcinoma: results from phase I/II studies. J Clin Oncol. 2004; 22:1815–1822. PMID: 15143074.


99. Ascierto PA, Scala S, Castello G, Daponte A, Simeone E, Ottaiano A, et al. Pegylated arginine deiminase treatment of patients with metastatic melanoma: results from phase I and II studies. J Clin Oncol. 2005; 23:7660–7668. PMID: 16234528.


100. Glazer ES, Piccirillo M, Albino V, Di Giacomo R, Palaia R, Mastro AA, et al. Phase II study of pegylated arginine deiminase for nonresectable and metastatic hepatocellular carcinoma. J Clin Oncol. 2010; 28:2220–2226. PMID: 20351325.


101. Ott PA, Carvajal RD, Pandit-Taskar N, Jungbluth AA, Hoffman EW, Wu BW, et al. Phase I/II study of pegylated arginine deiminase (ADI-PEG 20) in patients with advanced melanoma. Invest New Drugs. 2013; 31:425–434. PMID: 22864522.


102. Yang TS, Lu SN, Chao Y, Sheen IS, Lin CC, Wang TE, et al. A randomised phase II study of pegylated arginine deiminase (ADI-PEG 20) in Asian advanced hepatocellular carcinoma patients. Br J Cancer. 2010; 103:954–960. PMID: 20808309.


103. Szlosarek PW, Steele JP, Sheaff MT, Avril NE, Szyszko T, Ellis S, et al. A randomised phase II trial of pegylated arginine deiminase (ADI-PEG20) in patients with malignant pleural mesothelioma (MPM). In : 2013 World Conference on Lung Cancer; 2013 Oct 27-30; Sydney. Abstr no. MO09.02.
104. Yau T, Cheng PN, Chan P, Chan W, Chen L, Yuen J, et al. A phase 1 dose-escalating study of pegylated recombinant human arginase 1 (Peg-rhArg1) in patients with advanced hepatocellular carcinoma. Invest New Drugs. 2013; 31:99–107. PMID: 22426640.


105. Szlosarek PW, Luong P, Phillips MM, Baccarini M, Stephen E, Szyszko T, et al. Metabolic response to pegylated arginine deiminase in mesothelioma with promoter methylation of argininosuccinate synthetase. J Clin Oncol. 2013; 31:e111–e113. PMID: 23319692.


106. Stelter L, Fuchs S, Jungbluth AA, Ritter G, Longo VA, Zanzonico P, et al. Evaluation of arginine deiminase treatment in melanoma xenografts using (18)F-FLT PET. Mol Imaging Biol. 2013; 15:768–775. PMID: 23722880.


Fig. 1
Heterogeneity of argininosuccinate synthetase 1 (ASS1) expression in lung adenocarcinoma. Immunohistochemical staining (BD ASS1 antibody 1:500, BioGenex Super Sensitive Detection System) of a non-small cell lung cancer composed of a mixed population of ASS1 positive and negative tumor cells (×400).
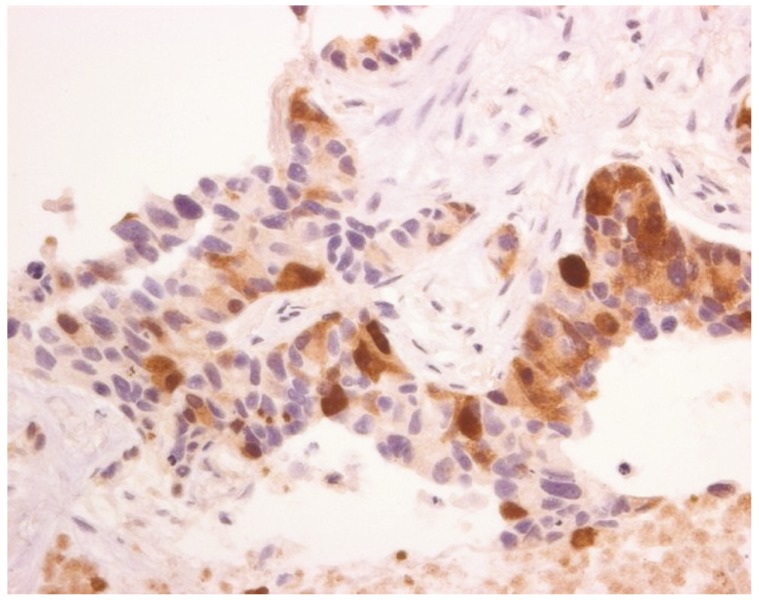
Fig. 2
Multiagent chemotherapy for arginine auxotrophic cancers. Applying the analogy of ten-pin bowling, a 'strike' is rarely seen in cancer therapy whereas a 'half-strike' is more likely with multiagent drug regimens; various drug combination studies are underway including the example shown here of the triplet, ADI-PEG20, cisplatin and pemetrexed. ASS1, argininosuccinate synthetase 1.

Table 1
Completed clinical studies of single-agent arginine depletors in advanced cancer
Study | No. of patients | Tumor type | Arginine depletor | Overall survival (mo) | Response rate (best response) | Comments |
---|---|---|---|---|---|---|
Izzo et al. (2004) [98] | 19 | HCC | ADI-PEG20 phase I/II | 13.7 | 47% (CR+PR) | Low toxicity |
Glazer et al. (2010) [100] | 76 | HCC | ADI-PEG20 phase II | 15.8a) | SD only | [Arginine] plasma reduced |
Yang et al. (2010) [102] | 71 | HCC | Randomized phase II: 160 or 320 IU/m2 of ADI-PEG20 | 7.3 | 31% (SD) | PFS 1.8 mo; heavily pre-treated patients |
Yau et al.(2013) [104] | 15 | HCC | peg-rhArg1 phase I | 5.1 | 26.7% (SD) >8 wk | PFS 2.8 mo; heavily pre-treated; OBD=1,600 IU/kg; I.V. administration |
Ascierto et al. (2005) [99] | 39 | Melanoma | ADI-PEG20 phase I/II | 15 | 25% (CR+PR) | Reduced NO synthesis consistent with MOA |
Feun et al. (2012) [33] | 38 | Melanoma | ADI-PEG20 phase II |
14.6 (ASS1-ve) vs. 9.3 (ASS1+ve) (p=0.374) |
11% (PR) in ASS1-ve only | PFS 3.6 mo (ASS1-ve) vs. 1.8 mo (ASS1+ve) (p=0.025); 74% ASS1-ve frequency; ASS1 re-expression on relapse (n=2/2) |
Ott et al. (2013) [101] | 31 | Melanoma | ADI-PEG20 phase I/II | n/a | 25% Early PMR, 29% (SD) | Prolonged SD in choroidal melanoma |
Szlosarek et al. (2013) [103,105] | 68 | Mesothelioma | Randomized phase II in ASS1-ve patients: ADI- PEG20+BSC (n=44) vs. BSC alone (n=24) | n/a | 46% Early PMR, SD by modified RECIST | PFS 3.3 mo vs. 1.9 mo (p=0.02) favoring ADI-PEG20+BSC; ASS1 methylation status correlated with IHC (p=0.025) |
HCC, hepatocellular carcinoma; CR, complete response; PR, partial response; SD, stable disease; PFS, progression-free survival; NO, nitric oxide; MOA, mechanism of action; ASS1, argininosuccinate synthetase 1; n/a, not available; PMR, partial metabolic response; OBD, optimal biological dose; BSC, best supportive care; RECIST, Response Evaluation Criteria in Solid Tumors; IHC, immunohistochemistry. a)From diagnosis.
Table 2
Phase I/II arginine depletor combination studies in advanced cancer