Abstract
An in vitro model for ischemia/reperfusion injury has not been well-established. We hypothesized that this failure may be caused by serum deprivation, the use of glutamine-containing media, and absence of acidosis. Cell viability of H9c2 cells was significantly decreased by serum deprivation. In this condition, reperfusion damage was not observed even after simulating severe ischemia. However, when cells were cultured under 10% dialyzed FBS, cell viability was less affected compared to cells cultured under serum deprivation and reperfusion damage was observed after hypoxia for 24 h. Reperfusion damage after glucose or glutamine deprivation under hypoxia was not significantly different from that after hypoxia only. However, with both glucose and glutamine deprivation, reperfusion damage was significantly increased. After hypoxia with lactic acidosis, reperfusion damage was comparable with that after hypoxia with glucose and glutamine deprivation. Although high-passage H9c2 cells were more resistant to reperfusion damage than low-passage cells, reperfusion damage was observed especially after hypoxia and acidosis with glucose and glutamine deprivation. Cell death induced by reperfusion after hypoxia with acidosis was not prevented by apoptosis, autophagy, or necroptosis inhibitors, but significantly decreased by ferrostatin-1, a ferroptosis inhibitor, and deferoxamine, an iron chelator. These data suggested that in our SIR model, cell death due to reperfusion injury is likely to occur via ferroptosis, which is related with ischemia/reperfusion-induced cell death in vivo. In conclusion, we established an optimal reperfusion injury model, in which ferroptotic cell death occurred by hypoxia and acidosis with or without glucose/glutamine deprivation under 10% dialyzed FBS.
Ischemia/reperfusion injury of myocardial cells is an important pathophysiology of ischemic heart disease, and occurs as a complication of open-heart surgery and cardiac transplantation. During ischemia, ATP levels decrease as the mitochondrial electron transport chain stops operating. Meanwhile, increased anaerobic glycolysis results in intracellular accumulation of protons and lactate [1]. As these alterations progress, irreversible cellular damage occurs. Prompt reperfusion is required to salvage the ischemic myocardium, but reperfusion after a significant duration of ischemia is accompanied by a paradoxical acceleration of cell death, known as reperfusion injury [2]. Reperfusion injury may be caused by multiple factors, such as sudden reintroduction of oxygen and abrupt normalization of interstitial pH and osmolarity, which subsequently results in oxidative stress and impaired sodium and calcium ion homeostasis [3].
Pathophysiologic mechanisms and potential therapeutic interventions for myocardial ischemia-reperfusion injury have been extensively explored utilizing diverse experimental models, including in vivo regional myocardial ischemia, global ischemia of the perfused heart, and numerous cardioplegia models. Meanwhile, many investigators sought to establish simulated ischemia/reperfusion (SIR) models using cultured cells, as these in vitro models enable specific manipulation of individual microenvironmental factors and eliminate confounding effects of non-myocardial cells. Moreover, using an immortalized cell line such as H9c2 cells bypasses the time consumption and low reproducibility of primary cardiac cell culture. In this context, we have also attempted to establish an SIR model using H9c2 cells and reported the effects of various microenvironmental factors on the outcome of SIR, especially the effects of lactic acidosis during simulated ischemia (SI) [4].
However, due to the complexity of microenvironmental changes during in vivo ischemia-reperfusion and the altered phenotypes of transformed cells, the reliability of SIR has been continuously questioned. In a recent report, Yang et al. [5] reviewed hundreds of SIR studies using the H9c2 cell line and, after selecting six representative SIR protocols, compared the effects of SI alone versus SIR on lactate dehydrogenase (LDH) release, ATP depletion, reactive oxygen species (ROS) generation, and other pathologies. Disappointingly, none of these models were reflective of the in vivo phenomenon, as cell death measured by LDH release progressed rapidly during SI, but was suppressed by subsequent simulated reperfusion (SR), failing to induce the most characteristic feature of ischemia-reperfusion, i.e., accelerated cell death during the early phase of reperfusion. Moreover, SR failed to induce ROS generation and impaired ATP repletion. Based on these results, Yang et al. [5] concluded that these models cannot simulate in vivo ischemia-reperfusion, and thus are not suitable for the study of myocardial ischemia/reperfusion.
Notwithstanding this conclusion, we recognized a prevailing mistake in these studies, including our own, which may mislead the experiments. In the most common SI protocols adopted thus far, cells were subjected to concomitant serum withdrawal, glucose deprivation and hypoxia to simulate ischemia. Among these three conditions, glucose deprivation and hypoxia are inherent to ischemia, but serum deprivation cannot be regarded as a natural consequence of ischemia. Unlike glucose and oxygen, serum constituents such as carrier molecules (e.g., albumin or transferrin) or signaling molecules (e.g., hormones or growth factors) are not thought to be depleted during a relatively short ischemic episode, thus arguing against the inclusion of serum withdrawal in simulation of ischemia. Moreover, serum withdrawal in most cultured cells triggers extensive cell death, which is mediated by increased mitochondrial ROS generation [6]. In fact, the study of Yang et al. [5] demonstrated that ROS levels were increased by SI, and subsequently decreased upon SR when the experiment was conducted under serum withdrawal conditions. These results demonstrated that the characteristic “oxygen paradox” phenomenon during reperfusion is perturbed by prior serum withdrawal.
Another common practice in SIR experiments is the utilization of Dulbecco's modified Eagle's media (DMEM) as the basic extracellular fluid. Contrastingly, most perfused heart studies use Krebs-Henseleit (KH) physiologic solution, which is different in many aspects from DMEM. DMEM includes many additional constituents not present in simple KH buffer, including glutamine. In most transformed cells, glutamine can serve as an alternative energy substrate under glucose deprivation. Moreover, numerous recent reports revealed that glutamine plays an important role in ischemic and reperfused myocardial cells. In fact, glutamine exerted a protective effect against ATP depletion and cell damage during ischemia, serving as an alternative energy substrate [7]. On the other hand, glutaminolysis is a critical inducer of ferroptosis, a specialized form of iron-dependent necrotic cell death. Currently ferroptosis has been supposed to be a major mechanism of cell death in ischemia-reperfusion injury, and inhibition of glutaminolysis under nutrient deprivation can reduce myocardial injury triggered by ischemia-reperfusion [8]. Because DMEM contains a supraphysiologic concentration of glutamine (4 mM), it is probable that the presence of glutamine in DMEM would significantly affect the outcome of SIR, which should be taken into consideration in design of SIR experiments and interpretation of results.
Therefore, we attempted to clarify the effects of serum deprivation and glutamine on SIR using H9c2 cell line, and establish an optimal SIR model based on these results.
The H9c2 cell line was purchased from ATCC. Fetal bovine serum (FBS) was purchased from Gibco (Grand Island, NY, USA). Low glucose Dulbecco's modified Eagle's media (DMEM) used to maintain the cell line and simulate reperfusion was purchased from Hyclone (Catalog number 30021.01) (Logan, UT, USA). DMEM used in hypoxia conditions was purchased from Sigma Aldrich (Catalog number D5030) (St. Louis, MO, USA). SnakeSkin pleated dialysis tubing was purchased from Thermo Scientific (Waltham, MA, USA). Cell culture dishes were purchased from Falcon (Oxnard, CA, USA). Other reagents were obtained from Sigma Aldrich.
H9c2 cells, an immortalized cell line derived from rat embryonic cardiac myoblasts, were initially cultured as monolayers in DMEM supplemented with 10% FBS. Cells were grown under an atmosphere of 5% CO2 at 37℃. We seeded 300,000 cells in T75 flasks and 60,000 cells in 35 mm dishes on Monday. Cells grown at T75 flasks underwent a media change on Wednesday, were subcultured on Thursday, and 300,000 cells were seeded again in a T75. A media change was performed on Saturday, and cells were subcultured again on Monday. Cells grown in 35 mm dishes were subjected to a media change on Wednesday, and were used for experiments on Friday (Fig. 1).
To induce hypoxia, 37℃, 1% O2 and 5% CO2 gas phase was prepared using a Galaxy 48 R incubator (Eppendorf, Hamburg, Germany), and cells were cultured in these conditions for 24 h. In the control group, 24 mM sodium bicarbonate was added to DMEM powder (D5030) to adjust the medium pH to 7.4. In the acidosis group (lactic acidosis), 2.4 mM Na-bicarbonate and 21.6 mM Na-lactate were added to adjust the medium pH to 6.4.
Media were pre-incubated for 3–4 h in an incubator prior to use.
SnakeSkin pleated dialysis tubing was filled with 110 ml FBS, and tubes were sealed on both sides and placed in 4 L PBS. This preparation was continuously stirred in a cold room using a magnetic stirrer. PBS was replaced with fresh PBS every 12 h. FBS dialysis was performed for 3 days.
For preparation of the reaction buffer, thiazolyl blue tetrazolium bromide was dissolved in PBS at a concentration of 5 mg/ml and mixed at a ratio of 1:9 with DMEM containing 10% FBS. Medium used for cell culture was removed. After washing twice with PBS, reaction buffer was treated. After two hours, media was removed, formazan was dissolved using DMSO, and absorbance was measured at 562 nm.
Medium was collected and centrifuged for five minutes at 21,000 G. Cell lysate was collected in 1 ml PBS containing 0.2% triton X-100, and centrifuged for five minutes at 21,000 G. Cell lysate was diluted 1:3 with PBS. 160 µl of reaction buffer containing 156 µl of 100 mM potassium phosphate buffer (pH 7.4), 2 µl 18 mM NADH and 2 µl 60 mM sodium pyruvate was mixed with 40 µl medium or cell lysate sample. NADH was measured by spectrophotometry at 340 nm for 100 sec, and the concentration change per minute was calculated to estimate LDH activity.
In the figures, LDH release during reperfusion (%) was calculated by dividing the amount of medium LDH collected after reperfusion by the sum of the amount of medium and cell lysate LDH collected after reperfusion. In the results section, LDH release during ischemia was calculated by dividing the amount of medium LDH collected after ischemia by the sum of the amount of cell lysate LDH prepped after reperfusion and medium collected after ischemia and reperfusion.
In many experiments to simulate ischemia-reperfusion, investigators have lowered FBS to 0% or 1%. Under in vivo ischemic conditions, many substances in the serum are expected to be depleted, such as glucose and oxygen. Therefore, many investigators have considered serum deprivation to be a basic condition of the ischemia model. However, considering the typical clinical situation, in which percutaneous coronary intervention (PCI) for reperfusion is performed within a short time after complete obstruction of the coronary arteries, the duration of ischemia is generally less than four hours in patients with reperfusion injury [910]. For this duration of ischemia, serum components such as growth factors and hormones are unlikely to be depleted. Therefore, we speculated that serum deprivation may not be necessary or relevant for in vitro models of ischemia/reperfusion injury as serum deprivation per se would induce a significant cell death. Therefore, we conducted an experiment to determine the effects of serum deprivation on H9c2 cell viability. Cell count and viability in H9c2 cells grown in 10%, 1%, and 0% FBS for 24 h was evaluated using an MTT assay.
Compared with 10% FBS, viable cell was about 75% when 1% FBS was used, and about 57% when 0% FBS was used. This suggested that FBS deprivation alone had a significant effect on cell viability or cell proliferation. When 10% dialyzed FBS was supplemented, viable cell was approximately 85% relative to cells supplemented with 10% normal FBS. However, 10% dialyzed FBS was highly effective in protecting cells from serum deprivation (Fig. 2).
SIR experiments are generally conducted without addition of FBS. However, it was considered inappropriate to conduct experiments in a 0% FBS environment, where viable cells were significantly decreased to 40% within 24 h. Therefore, we tried 1% FBS as serum deprivation conditions. To adapt the cells to the 1% FBS environment, cells were preincubated in DMEM containing 1% FBS for 11 h prior to the experiment, and subsequently subjected to SIR.
Twenty-four hours after cells were cultured under hypoxia alone or hypoxia with glucose and glutamine deprivation and acidosis, reperfusion was simulated by replacing media with full DMEM containing 1% FBS under normoxia. Seventeen hours after the media change, LDH release was not increased in SIR models compared to cells grown in normoxia with DMEM containing 1% serum. There was no significant difference in cell density evidenced by cell photographs. In conclusion, cell damage caused by reperfusion was not observed in 1% serum conditions (Fig. 3).
Generally, glucose deprivation is performed in experiments simulating ischemia. However, glutamine is an amino acid that can be used as an energy substrate. Therefore, glutamine may alleviate the effects of glucose deprivation. In order to investigate the effect of glutamine on glucose deprivation, we evaluated the effects of glucose deprivation versus glucose and glutamine deprivation.
24 mM sodium bicarbonate was added to the DMEM powder (D5030 from Sigma-Aldrich), and the medium was prepared by adding glucose and/or glutamine. Ischemic damage was estimated by measuring LDH release in glucose or glucose/glutamine deprivation conditions in a hypoxic environment for 24 h. At the end of ischemic simulation, medium was changed to low-glucose DMEM in a normoxic environment, and medium and cellular LDH were measured 17 h later to assess reperfusion damage.
In normoxia, there was no significant difference in cell death between the glucose deprivation group and the glutamine deprivation group (about 10%). In the glucose/glutamine deprivation group, about 95% of the cells died in 24 h. In hypoxia, LDH release was about 15% in the glucose deprivation group, about 5% in the glutamine deprivation group, and about 45% ± 21% in the glucose/glutamine deprivation group (data not shown).
When hypoxic stress was induced in cells receiving DMEM containing 10% dialyzed FBS, LDH release increased after reoxygenation. There was no additive effect of glucose deprivation or glutamine deprivation during hypoxia when LDH release was measured 17 h after medium change for SR. However, in the glucose/glutamine deprivation group, LDH release was significantly increased during reperfusion compared with not only the control group, but also with the glucose deprivation group and glutamine deprivation group (Fig. 4).
These findings suggested that glutamine deprivation did not affect cell damage during hypoxia, but that glutamine supplementation in ischemia protected cells from reperfusion damage following hypoxia with glucose deprivation. These results indicated that glutamine as well as glucose should be depleted during ischemia in order to induce maximal reperfusion damage.
Ischemic acidosis is well-known to cause cell damage after sudden pH normalization in reperfusion [11]. Therefore, we also performed an experiment to investigate whether acidosis during hypoxia contributes to reperfusion injury in vitro.
Cells cultured for 24 h in acidosis released about 8% LDH in normoxic or hypoxic conditions, which did not significantly differ from LDH release in the control group. However, LDH release measured 17 h after the media change to simulate reperfusion was significantly increased in the hypoxia and acidosis group compared to the hypoxia alone group. Although there was no significant difference in LDH release between the acidosis group and the acidosis with glucose/glutamine deprivation group, nor between the acidosis group and the glucose/glutamine deprivation group under hypoxia, an additive effect of acidosis was observed between the glucose/glutamine deprivation group and the acidosis with glucose/glutamine deprivation group under hypoxia (Fig. 5). These results suggested that hypoxia with acidosis, or hypoxia with acidosis and glucose/glutamine deprivation, were adequate to induce reperfusion damage in H9c2 cells.
During our experiments, we noticed that LDH release induced by reperfusion suddenly increased in comparison with the results of previous experiments. At that time, we used passage 3 cells, which had been frozen almost immediately upon receipt from ATCC. Therefore, reperfusion damage was tested in low-passage H9c2 versus high-passage H9c2 of passage 20 or higher.
High-passage cells were more resistant to reperfusion damage. Regardless of hypoxic conditions, overall reperfusion damage was lower. Particularly in cells cultured under hypoxia and acidosis conditions, reperfusion damage was significantly reduced and statistical significance was not observed compared to hypoxia alone. However, in the glucose/glutamine deprivation group, reperfusion damage was significantly higher compared to hypoxia alone, and the hypoxia, acidosis, and glucose/glutamine deprivation group showed significantly increased reperfusion damage compared to the hypoxia with acidosis group (Fig. 6). These data suggested that the degree of reperfusion damage was dependent on H9c2 cell passage, and that hypoxia, acidosis, and glucose/glutamine deprivation is the model which can be more widely used for a reperfusion injury model compared to hypoxia with acidosis.
In 2015, Gao et al. [8] reported that cardiac reperfusion injury is associated with ferroptosis. Since then, several additional groups have reported that cell death induced by reperfusion damage occurs via ferroptosis [121314]. Therefore, we examined the cell death mechanism in our SIR model to determine whether reperfusion damage is also related to iron-dependent cell death in this model, and to clarify how well our SIR model reflects in vivo ischemia/reperfusion injury.
We used hypoxia with acidosis as an ischemia model with cells below passage 15 to exclude the effect of glucose/glutamine deprivation on cell death. LDH release was approximately 51% ± 5% after reperfusion in the control group. LDH release was 57% ± 0.5% when we added 50 µM of the pan-caspase inhibitor z-VAD-FMK to inhibit apoptosis, and 54% ± 0.5% when we added 1 nM of bafilomycin A1 to inhibit autophagy, which was not statistically significant compared to that of the control group. In addition, LDH release was 50% ± 3%, or 58% ± 1% respectively, when treated with the necroptosis inhibitors necrostatin-1 or GSK'872, and there was no statistically significant difference from that of the control group. However, treatment with ferrostatin-1 or deferoxamine, which are widely used ferroptosis inhibitors, significantly reduced LDH release to 13% ± 2% and 20% ± 1.5%, respectively (Fig. 7). These data suggested that the majority of cell death induced by reperfusion in our SIR model, which was possibly ferroptosis.
In vivo studies of cardiac ischemia/reperfusion injury have been simulated by compressing the coronary artery. Several decades ago, equipment for studying the heart in organ units, such as Langendorff perfusion apparatus, was developed and used extensively in studies of ischemia/reperfusion injury. However, because in vivo ischemia/reperfusion studies are conducted in a complex system, there are many confounding factors that makes it difficult to identify the responsible mechanisms of action. Furthermore, organ studies are labor-intensive due to the maintenance of the apparatus, the surgery to obtain the heart, and so on. Equipment for organ studies and animals to obtain the heart are also costly. In vitro experiments are desirable, as confounding factors can easily be controlled, and these studies are simpler and less expensive to perform than in vivo or organ studies. Therefore, it is beneficial to establish an adequate in vitro ischemia/reperfusion model.
However, in vitro modeling of ischemia/reperfusion injury has not yet been established until now. This is due in part to inconsistency of ischemia and reperfusion conditions across investigators. Therefore, the conditions of ischemia and reperfusion must be taken into account when interpreting the results of in vitro ischemia/reperfusion studies. In most ischemia/reperfusion experiments conducted in vitro , apoptosis was observed rather than necrosis in cell death. However, since apoptosis also occurs under serum deprivation per se in H9c2 cells, it is difficult to discriminate between cell death induced by ischemia/reperfusion damage and cell death induced by serum deprivation [15]. Moreover, a recent effort to standardize this protocol failed, necessitating further investigations into a physiologically relevant in vitro model of ischemia/reperfusion [5].
We also tried to establish an ischemia/reperfusion model based on prior studies, and found that reperfusion damage was difficult to induce [4]. We ultimately questioned whether it is rational that we should study ischemia/reperfusion injury using serum-free media, which has been used in most in vitro models of ischemia/reperfusion injury. Serum deprivation during oxygen-glucose deprivation is a common practice, as serum contains nutrients such as glucose and glutamine. However, we speculated that conditions without compounds in serum, such as growth factors or hormones, were different from in vivo conditions.
In fact, serum deprivation alone had a significant effect on H9c2 cell viability. When cells were observed under a microscope, numerous cells appeared to be undergoing apoptosis (Fig. 2). In addition, when ischemia was performed with 1% FBS, reperfusion damage was not apparently present (Fig. 3). Serum deprivation affects cells in many ways, including cell death, cell cycle, and cell differentiation [1617]. The absence of reperfusion damage may be explained by cell death due to serum deprivation, which was severe enough to mask reperfusion damage. Alternatively, cell death due to reperfusion damage was suppressed by cell cycle arrest caused by serum deprivation, similar to normal cells being more resistant to anticancer agents than cancer cells. Another possibility is that the cell's characteristics were altered by serum deprivation. Further research is necessary to fully assess the effects of serum deprivation on reperfusion injury.
These results prompted us to use dialyzed FBS to filter out small substances such as glucose and glutamine, while leaving large molecular weight substances such as growth factors and hormones. When dialyzed FBS was used, cell viability was reduced compared to cells receiving full FBS. It was speculated that the loss of important serum components such as insulin, which are smaller than the cut-off size of the dialysis membrane, might have affected cell survival. However, cell viability was higher compared to the serum deprivation group (Fig. 2). We confirmed that reperfusion injury can be observed when H9c2 cells were cultured using dialyzed FBS during ischemia simulation. Therefore, we suggest using a 10% mixture of dialyzed FBS in DMEM (D5030) while maintaining the cells in hypoxia conditions as an ischemic model.
We next investigated the effect of acidosis and glucose/glutamine deprivation on reperfusion-induced cell damage following ischemia. LDH release increased dramatically within 17 h of medium exchange to simulate reperfusion after ischemic simulations in which cells were cultured under hypoxic conditions with or without acidosis or glutamine/glucose deprivation for 24 h (Figs. 4 and 5). Although reperfusion injury was not apparent in the hypoxia-only group compared to the hypoxia and acidosis group, hypoxia alone could also induce reperfusion injury (Figs. 4 and 5). However, in order to increase reperfusion damage, it is recommended to incubate cells in a hypoxic environment with acidosis conditions induced by dissolving NaHCO3 in DMEM at a concentration of 2.4 mM, which results in pH 6.4 under 5% carbon dioxide. In addition, if acidosis with hypoxia alone is not effective to induce reperfusion damage, it would be possible to observe reperfusion damage by adding glucose/glutamine deprivation conditions (Figs. 4 and 5). Although these findings lay groundwork for in vitro modeling of ischemia/reperfusion injury, many questions remain. First, further studies are needed to fully assess whether the model developed here is physiologically relevant to in vivo ischemia/reperfusion injury. It is also unclear why reperfusion damage was not observed under the condition of 1% serum. Indeed, it is likely that reperfusion damage in clinical practice is likely to be associated with certain components of the serum.
Reperfusion damage was decreased when glucose or glutamine was present during simulated ischemia. It is speculated that glucose protected cells by supplying ATP or NADPH, which is a key molecule in the antioxidant system [18]. As glutamine is also a source of ATP [1920] and NADPH [2122], it was speculated that glutamine would replace glucose in the absence of glucose. In fact, under normoxia, cell death did not increase when either glucose or glutamine was deprived. However, in case of glutamine, there are incompatible reports that glutamine reduces ischemia-reperfusion injury [7] and that glutaminolysis increases ischemia-reperfusion damage [8]. Given that it was reported glutaminolysis played an important role in lipid peroxidation [23], we speculated glutamine during ischemia possibly has a cell protection effect, but glutamine during reperfusion is a source of ROS through glutaminolysis.
In normoxia, over 95% of cells died when subjected to glutamine/glucose deprivation, whereas in hypoxia, 67% ± 12% of cells died by glutamine/glucose deprivation. These data suggested that oxygen promoted glucose/glutamine deprivation-induced cell death through an as yet undetermined mechanism. Some patients with trauma or compartment syndrome receive hyperbaric oxygen therapy (HBOT) to kill microorganisms, reduce edema, or supply tissue oxygen. However, compartment syndrome generally accompanies ischemia, suggesting that tissues are also subject to glucose deprivation [24]. Selecting the appropriate patients to receive HBOT will increase the therapeutic effectiveness of HBOT. Elucidating the mechanism by which oxygen increases glutamine/glucose deprivation-induced cell death is expected to contribute to identification of suitable patients for HBOT.
Acidosis alone did not increase LDH release in normoxia or hypoxia. Further, acidosis in normoxia did not induce cell death even when abrupt pH normalization occurred. However, after acidosis and hypoxia, there was a synergistic effect on LDH release with sudden reoxygenation and pH normalization (Fig. 5). In fact, there are several reports suggesting that slowly restoring acidosis in vivo reduced reperfusion damage [2526]. Therefore, these data implied that our SIR model is reflective of in vivo ischemia-reperfusion injury. Studies of mitochondrial dynamics in hypoxia or acidosis are currently underway [27]. Our SIR model appears to be a suitable model for studying the effect of mitochondrial dynamics on cell survival in ischemia/reperfusion.
Reperfusion-induced cell death is likely to occur via ferroptosis in our SIR model. Specifically, we found that ferrostatin-1 and deferoxamine, which are widely used ferroptosis inhibitors, significantly reduce reperfusion damage (Fig. 7). Recent reports have demonstrated that ferroptosis occurs in cardiac ischemia/reperfusion injury [8]. In fact, several clinical studies demonstrated that treating with deferoxamine after reperfusion reduced the cardiac infarct size [2829]. Therefore, these data also suggested that our SIR model is reflective of myocardial infarction. In addition, current studies of ferroptosis are limited in scope, as ferroptosis must be artificially induced with erastin or RSL-3 [30]. Our SIR model may be used as a pathophysiologic ferroptosis model.
Our SIR model induced reperfusion damage not only in H9c2 cells, but also in Sk-hep1 cells (data not shown). Depending on cell characteristics, the degree of reperfusion-induced cell death may differ (Fig. 6), but this phenomenon is potentially ubiquitous and not limited to H9c2 cells. Although cell characteristics must be taken into account, our SIR model may be helpful for establishing in vitro ischemia reperfusion models for liver, kidney, or neurons. Further research is needed to determine whether our SIR model will also be helpful in studying other organs.
In conclusion, reperfusion injury can be induced in vitro by media change after incubation under hypoxia and acidosis with or without glutamine/glucose deprivation in DMEM containing 10% dialyzed FBS.
Figures and Tables
Fig. 1
Experimental schedule.
SI stands for simulated ischemia and SR stands for simulated reperfusion. SI, simulated ischemia; SR, simulated reperfusion.
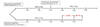
Fig. 2
Effect of fetal bovine serum (FBS) deprivation and 10% dialyzed FBS on H9c2 cell viability.
To investigate the effect of serum deprivation and 10% dialyzed FBS on cell viability, MTT assay was performed. H9c2 cells were seeded in 6-well plates at 60,000 cells per well and cultured for four days. A media change was carried out once two days after seeding. After the medium was removed and cells were washed twice with PBS, DMEM was changed with FBS or dialyzed FBS at the concentration of the above experimental conditions. After culturing for 24 h, the medium was removed and 0.5 mg/ml Thiazolyl Blue Tetrazolium Blue (MTT) powder suspended in DMEM containing 10% FBS was treated. After 2 h, medium was removed, and cells were washed twice with PBS and subsequently treated with DMSO. The formazan solution was transferred to a 96-well plate and absorbance was measured at 562 nm. Data are expressed as means ± S.D. (n = 3) (**p < 0.01 vs. 10% FBS, and #p < 0.05 or ##p < 0.01 vs. 10% diFBS).
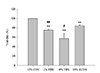
Fig. 3
Reperfusion damage under 1% serum condition.
Ischemia/reperfusion simulation was performed under 1% fetal bovine serum (FBS) conditions. The control group was cultured in DMEM containing 1% FBS for 24 h under normoxia. The hypoxia group was cultured for 24 h under hypoxia (1% oxygen). To simulate extreme ischemia, cells were cultured under hypoxia, glucose/glutamine deprivation (GGD), and lactic acidosis (pH 6.4). Cells were cultured for 24 h in these conditions. After the ischemic period, the medium was changed with DMEM containing 1% FBS and incubated under normoxia. Seventeen hours after the media change, lactate dehydrogenase (LDH) release was measured. Cell photographs were taken as soon as ischemia was over and as soon as the reperfusion was over. Data are expressed as means ± S.D. (n = 3). Scale bar: 200 µm.
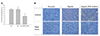
Fig. 4
Under 10% dialyzed fetal bovine serum (FBS), reperfusion damage was significantly increased when both glucose and glutamine were deprived.
SnakeSkin wrinkle dialysis tubing was used to remove material ≤ 10 kDa in FBS, including nutrients such as glucose and glutamine. Dialyzed FBS was used for ischemia simulation with DMEM at a concentration of 10%. After culturing for 24 h under the specified conditions, cells were photographed. The medium was changed with DMEM containing 10% FBS. After 17 h incubation under normoxia, cells were photographed and lactate dehydrogenase (LDH) release was measured. Cell passage used in these experiments was below 20. Data are expressed as means ± S.D. (n = 3) (++p < 0.01 vs. control under normoxia, **p < 0.01 vs. control under hypoxia, #p < 0.05 vs. glucose/glutamine deprivation under hypoxia). Scale bar: 200 µm.
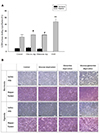
Fig. 5
Acidosis during hypoxia significantly induced reperfusion damage.
To produce media with pH 6.4 under 5% CO2, 2.4 mM NaHCO3, obtained from the Henderson-Hasselbach equation, was added to DMEM (D5030). In the control group, 24 mM NaHCO3 was added to achieve pH 7.4. Therefore, we added 21.6 mM Na-lactate to match the osmolality of the media used to induce acidosis in the control group. As a result, we used the media, which is made for simulating lactic acidosis. Media pH was measured 7.4–7.5 in the control group and 6.4–6.5 in the acidosis group. Cell passage used in these experiments was below 20. Data are expressed as means ± S.D. (n = 3) (*p < 0.05 and **p < 0.01vs. control under hypoxia, #p < 0.05 vs. glucose/glutamine deprivation + acidosis under hypoxia). GGD, glucose/glutamine deprivation; N.S., not significant. Scale bar: 200 µm.
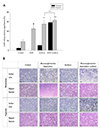
Fig. 6
High passage H9c2 cells obtained resistance to reperfusion damage.
SIR was performed using high-passage H9c2 cells grown for 5 months or more. The passage used in the high passage data in (A) above was 25–29, and the passage of H9c2 used in the photograph of (B) was 27. Cells were maintained by subculturing twice weekly (Monday, Thursday). During subculturing, cell density did not exceed 90%, and media changed were also performed periodically (Wednesday, Saturday). There were no special events to mention while we maintain cells, such as extensive cell death or sudden changes in cell number. Data are expressed as means ± S.D. (high passage n = 4, low passage n = 3) (**p < 0.01 vs. low passage, ##p < 0.01vs. acidosis with hypoxia). LDH, lactate dehydrogenase; SIR, simulated ischemia/reperfusion; GGD, glucose/glutamine deprivation. Statistical analyses were conducted using an unpaired t-test. Scale bar: 200 µm.
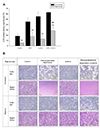
Fig. 7
Reperfusion induced iron-dependent cell death, which was likely ferroptosis.
Before the media change to simulate the reperfusion, a media change was performed after adjusting the temperature and pH by incubating for 3–4 h in the incubator. At this time, 100 µM deferoxamine, 1 µM ferrostatin-1, and 20 µM necrostatin-1 were added to the media before incubation. Data are expressed as means ± S.D. (n = 3) (*p < 0.05 and **p < 0.01 vs. control). LDH, lactate dehydrogenase. Scale bar: 200 µm.
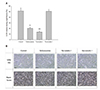
ACKNOWLEDGEMENTS
This research was supported by Basic Science Research Program through the National Research Foundation of Korea (NRF) funded by the Ministry of Education (NRF-2018R1C1B6003879) and a National Research Foundation of Korea (NRF) MRC grant (2018R1A5A2020732).
Notes
Author contributions E.S. contributes to making conception and design, analyzing data, writing the manuscript, and revising it; D.L. contributes to acquisition and analysis of data; C.W.W. contributes to funding acquisition, making conception, and providing resources; Y.H.K. contributes to making conception and design, analyzing data, writing the manuscript, revising it, and supervising the research.
References
1. King LM, Opie LH. Glucose and glycogen utilisation in myocardial ischemia--changes in metabolism and consequences for the myocyte. Mol Cell Biochem. 1998; 180:3–26.

3. Hausenloy DJ, Yellon DM. Myocardial ischemia-reperfusion injury: a neglected therapeutic target. J Clin Invest. 2013; 123:92–100.



4. Lee JW, Lee HK, Kim HW, Kim YH. Effects of pH, buffer system and lactate on the simulated ischemia-reperfusion injury of H9c2 cardiac myocytes. Korean J Physiol Pharmacol. 2007; 11:45–55.
5. Yang GZ, Xue FS, Liu YY, Li HX, Liu Q, Liao X. Feasibility analysis of oxygen-glucose deprivation-nutrition resumption on H9c2 cells in vitro models of myocardial ischemia-reperfusion injury. Chin Med J (Engl). 2018; 131:2277–2286.


6. Lee SB, Kim JJ, Kim TW, Kim BS, Lee MS, Yoo YD. Serum deprivation-induced reactive oxygen species production is mediated by Romo1. Apoptosis. 2010; 15:204–218.


7. Khogali SE, Harper AA, Lyall JA, Rennie MJ. Effects of L-glutamine on post-ischaemic cardiac function: protection and rescue. J Mol Cell Cardiol. 1998; 30:819–827.

8. Gao M, Monian P, Quadri N, Ramasamy R, Jiang X. Glutaminolysis and transferrin regulate ferroptosis. Mol Cell. 2015; 59:298–308.



9. Steg PG, James SK, Gersh BJ. evidence-based recommendations, ensuring optimal patient management. Heart. 2013; 99:1156–1157.

11. Bond JM, Herman B, Lemasters JJ. Protection by acidotic pH against anoxia/reoxygenation injury to rat neonatal cardiac myocytes. Biochem Biophys Res Commun. 1991; 179:798–803.


12. Li L, Hao Y, Zhao Y, Wang H, Zhao X, Jiang Y, Gao F. Ferroptosis is associated with oxygen-glucose deprivation/reoxygenation-induced Sertoli cell death. Int J Mol Med. 2018; 41:3051–3062.


13. Li Y, Feng D, Wang Z, Zhao Y, Sun R, Tian D, Liu D, Zhang F, Ning S, Yao J, Tian X. Ischemia-induced ACSL4 activation contributes to ferroptosis-mediated tissue injury in intestinal ischemia/reperfusion. Cell Death Differ. 2019; 26:2284–2299.



14. Fang X, Wang H, Han D, Xie E, Yang X, Wei J, Gu S, Gao F, Zhu N, Yin X, Cheng Q, Zhang P, Dai W, Chen J, Yang F, Yang HT, Linkermann A, Gu W, Min J, Wang F. Ferroptosis as a target for protection against cardiomyopathy. Proc Natl Acad Sci U S A. 2019; 116:2672–2680.



15. Bonavita F, Stefanelli C, Giordano E, Columbaro M, Facchini A, Bonafè F, Caldarera CM, Guarnieri C. H9c2 cardiac myoblasts undergo apoptosis in a model of ischemia consisting of serum deprivation and hypoxia: inhibition by PMA. FEBS Lett. 2003; 536:85–91.


16. Kues WA, Anger M, Carnwath JW, Paul D, Motlik J, Niemann H. Cell cycle synchronization of porcine fetal fibroblasts: effects of serum deprivation and reversible cell cycle inhibitors. Biol Reprod. 2000; 62:412–419.

17. Howard MK, Burke LC, Mailhos C, Pizzey A, Gilbert CS, Lawson WD, Collins MK, Thomas NS, Latchman DS. Cell cycle arrest of proliferating neuronal cells by serum deprivation can result in either apoptosis or differentiation. J Neurochem. 1993; 60:1783–1791.


18. Jain M, Cui L, Brenner DA, Wang B, Handy DE, Leopold JA, Loscalzo J, Apstein CS, Liao R. Increased myocardial dysfunction after ischemia-reperfusion in mice lacking glucose-6-phosphate dehydrogenase. Circulation. 2004; 109:898–903.


19. Le A, Lane AN, Hamaker M, Bose S, Gouw A, Barbi J, Tsukamoto T, Rojas CJ, Slusher BS, Zhang H, Zimmerman LJ, Liebler DC, Slebos RJ, Lorkiewicz PK, Higashi RM, Fan TW, Dang CV. Glucose-independent glutamine metabolism via TCA cycling for proliferation and survival in B cells. Cell Metab. 2012; 15:110–121.


20. Fan J, Kamphorst JJ, Mathew R, Chung MK, White E, Shlomi T, Rabinowitz JD. Glutamine-driven oxidative phosphorylation is a major ATP source in transformed mammalian cells in both normoxia and hypoxia. Mol Syst Biol. 2013; 9:712.



21. DeBerardinis RJ, Mancuso A, Daikhin E, Nissim I, Yudkoff M, Wehrli S, Thompson CB. Beyond aerobic glycolysis: transformed cells can engage in glutamine metabolism that exceeds the requirement for protein and nucleotide synthesis. Proc Natl Acad Sci U S A. 2007; 104:19345–19350.



22. Son J, Lyssiotis CA, Ying H, Wang X, Hua S, Ligorio M, Perera RM, Ferrone CR, Mullarky E, Shyh-Chang N, Kang Y, Fleming JB, Bardeesy N, Asara JM, Haigis MC, DePinho RA, Cantley LC, Kimmelman AC. Glutamine supports pancreatic cancer growth through a KRAS-regulated metabolic pathway. Nature. 2013; 496:101–105.



23. Gao M, Yi J, Zhu J, Minikes AM, Monian P, Thompson CB, Jiang X. Role of Mitochondria in Ferroptosis. Mol Cell. 2019; 73:354–363.e3.


24. Myers RA. Hyperbaric oxygen therapy for trauma: crush injury, compartment syndrome, and other acute traumatic peripheral ischemias. Int Anesthesiol Clin. 2000; 38:139–151.


25. Kitakaze M, Weisfeldt ML, Marban E. Acidosis during early reperfusion prevents myocardial stunning in perfused ferret hearts. J Clin Invest. 1988; 82:920–927.



26. Inserte J, Barba I, Hernando V, Abellán A, Ruiz-Meana M, Rodríguez-Sinovas A, Garcia-Dorado D. Effect of acidic reperfusion on prolongation of intracellular acidosis and myocardial salvage. Cardiovasc Res. 2008; 77:782–790.


27. Khacho M, Tarabay M, Patten D, Khacho P, MacLaurin JG, Guadagno J, Bergeron R, Cregan SP, Harper ME, Park DS, Slack RS. Acidosis overrides oxygen deprivation to maintain mitochondrial function and cell survival. Nat Commun. 2014; 5:3550.



28. Williams RE, Zweier JL, Flaherty JT. Treatment with deferoxamine during ischemia improves functional and metabolic recovery and reduces reperfusion-induced oxygen radical generation in rabbit hearts. Circulation. 1991; 83:1006–1014.

