Abstract
Objective
To demonstrate the feasibility of using cardiothoracic CT for quantification of the initial right ventricle (RV) dimensions in infants with congenital heart disease (CHD) and a hypoplastic RV and to compare these measurements with those obtained in a control group with CHD without a hypoplastic RV.
Materials and Methods
Initial RV dimensions, including RV volumes, RV/left ventricle (LV) volume ratios, atrioventricular valve annulus diameter ratios, and RV/LV length ratios based on CT data, were collected from 57 infants with CHD and a hypoplastic RV (hypoplastic RV group; age range, 1 day to 6 months) and 33 infants with tetralogy of Fallot (control group; age range, 1 day to 6 months) and compared between the 2 groups. The type of final surgery was also evaluated in the hypoplastic RV group over a follow-up period of 3–8 years.
Results
The RV and LV volumes and lengths were successfully quantified in all 90 patients. The tricuspid valve annulus diameter could not be measured in cases showing muscular tricuspid atresia and double-inlet LV. The initial RV dimensions quantified by CT were significantly lower for the hypoplastic RV group than for the control group (p < 0.001). The types of final surgery performed in the hypoplastic RV group were univentricular repair in 46 patients, biventricular repair in 4 patients, or an indeterminate surgery in 7 patients.
In patients with congenital heart disease (CHD) and a hypoplastic right ventricle (RV), treatment strategies are generally divided into univentricular or biventricular repair depending on whether the RV can support the corresponding great arterial circulation (123456). Therefore, various echocardiographic measures have been used to quantify the initial RV size to predict the optimal treatment strategy for each patient (123456). Among these, the atrioventricular valve (AVV) index, RV/left ventricle (LV) length ratio, and tricuspid valve Z-score have been commonly used in clinical practice. In contrast to the aforementioned measures, which reflect only one component each, measurements of the RV volume itself may take into consideration all 3 anatomical components of the RV, i.e., the inlet, trabecular, and outlet portions. However, echocardiography shows considerably limited ability to accurately quantify the RV volume.
Recently, CT ventricular volumetry using a three-dimensional (3D) threshold-based segmentation approach was introduced, which can measure ventricular volumes with high accuracy and reproducibility in patients with CHD (7). Furthermore, the same methodology has been successfully applied for quantification of a hypoplastic LV volume (8910). However, CT ventricular volumetry has not yet been used to quantify a hypoplastic RV volume in patients with CHD. Therefore, this study aimed to demonstrate the feasibility of using cardiothoracic CT for quantification of initial RV dimensions in infants with CHD and a hypoplastic RV, and to compare them with those in a control group of infants with CHD but without a hypoplastic RV.
Approval for this retrospective study was obtained from the local Institutional Review Board and the requirement for informed consent was waived.
Between July 2011 and July 2017, 57 infants with CHD and a hypoplastic RV (median age at initial cardiothoracic CT, 7 days; range, 1 day to 6 months; M:F = 25:32) who underwent electrocardiography (ECG)-synchronized cardiothoracic CT examination before RV decompression therapy were included in this study (hypoplastic RV group). Cardiac defects in this group included tricuspid atresia (n = 22), pulmonary atresia with intact ventricular septum or critical pulmonary stenosis (n = 16), unbalanced atrioventricular septal defect (n = 2), and other types of functional single ventricle (n = 17) such as double-inlet LV, transposition of the great arteries with ventricular septal defect, and pulmonary atresia with ventricular septal defect. In cases where patients underwent 2 cardiothoracic CT examinations before RV decompression therapy, only the initial examination performed either preoperatively (n = 40) or after a palliative procedure (n = 17) was considered for this study. For the palliative procedure, pulmonary artery banding (n = 7), atrial septostomy or septectomy (n = 7), Blalock-Taussig shunt (n = 6), patent ductus arteriosus division or ligation (n = 6), central shunt (n = 2), ductal stenting (n = 1), aortic arch repair (n = 1), or right atrial reduction plasty (n = 1) were performed.
During the same period, 33 infants with tetralogy of Fallot (median age at initial cardiothoracic CT, 2.6 months; range, 1 day to 6 months; M:F = 15:18) who underwent ECG-synchronized cardiothoracic CT examination before total correction were assigned to the control group. Patients with tetralogy of Fallot who had a concomitant major cardiac defect potentially affecting the size of the RV, such as an atrioventricular defect, double-outlet RV, or absent pulmonary valve syndrome, were excluded from this study.
The body surface area on the cardiothoracic CT was obtained from the patients' electronic medical records. In the hypoplastic RV group, the type of final surgery, which was either univentricular or biventricular repair, was also reviewed. If a patient did not survive or a follow-up assessment was not performed before the final surgery, the type of final surgery was classified as indeterminate.
Cardiothoracic CT was performed using a secondgeneration dual-source scanner (SOMATOM Definition Flash; Siemens Healthineers, Forchheim, Germany) in all 90 patients. Fundamental CT scan parameters were as follows: 64 × 0.6-mm slices; gantry rotation time, 0.28 seconds; temporal resolution, 75 ms; slice width, 0.75 mm; and reconstruction interval, 0.4 mm. Radiation dose and image quality in CT imaging were optimized by positioning the patient in the isocenter of the CT gantry and by using combined tube current modulation (CARE Dose 4D; Siemens Healthineers), an individual body size-adapted radiation dose before scan range adjustment, and a sinogram-affirmed iterative reconstruction (SAFIRE; Siemens Healthineers) with a medium smooth kernel (I26f), and strength 5 (111213141516). To ensure greater radiation dose efficiency and iodine contrast-to-noise ratio, a 70 kV was used in all 90 patients. For ECG-synchronized data acquisition, prospectively ECG triggered sequential scanning was used in all 90 patients.
In the hypoplastic RV group, CT scanning was performed at end-systolic (ES) phase in 36 patients, at both ES and end-diastolic (ED) phases in 20 patients, and at the ED phase for 1 patient. In the control group, CT scanning was performed at both ES and ED phases in all 33 patients. To reduce respiratory motion artifacts, additional respiratory triggering using a respiratory gating system (AZ-733V; Anzai Medical Co, Tokyo, Japan) was applied in infants weighing more than 5 kg (17). This was applied in 10 patients in the hypoplastic RV group and 16 patients in the control group.
Iodinated contrast agent (Iomeron 400; iomeprol 400 mg I/mL; Bracco Imaging SpA, Milan, Italy; 1.5–2.0 mL/kg) was administered intravenously using a dual-head power injector at an injection rate of 0.3–0.8 mL/s according to body size. A triphasic or quadriphasic injection protocol was used to achieve uniform cardiovascular enhancement and minimal peri-venous streak artifacts. The scan delay time was determined using a bolus tracking technique with a trigger threshold of 150 Hounsfield units in the left ventricular cavity. For sedation, oral chloral hydrate (50 mg/kg) was administered initially, followed by intravenous midazolam (0.1 mg/kg) or ketamine (1 mg/kg) as required.
The reconstructed cardiothoracic CT image data were evaluated using a commercially available workstation (Advantage Workstation 4.6; GE Healthcare, Milwaukee, WI, USA). A semiautomatic 3D threshold-based segmentation method was used for ventricular volumetry, allowing for the exclusion of the papillary muscles and trabeculations from the ventricular cavity. An optimal threshold was individually determined to separate the most compact interventricular septal myocardium from the adjacent ventricular blood, following which the atrioventricular and semilunar valve planes were manually adjusted (18). The measured volumes of the RV and the LV were normalized to the body surface area, and the RV/LV volume ratio was subsequently calculated.
On a four-chamber CT image, the annulus diameters of the tricuspid and mitral valves were measured, and the AVV annulus diameter ratio was subsequently calculated (= tricuspid valve annulus diameter/mitral valve annulus diameter). On the same four-chamber CT image, the lengths of the RV and the LV were measured between the midpoint of the corresponding AVV valve annulus and the corresponding ventricular apex. Next, the RV/LV length ratio was calculated.
Continuous variables were presented as mean ± standard deviation or median and range, and categorical variables were expressed as a frequency (percentage). The Kolmogorov-Smirnov test was performed to determine a normal distribution. To analyze the statistical significance in the mean differences between the hypoplastic RV group and control group, an independent-sample nonparametric test was used for variables that did not fit into the normal distribution and an unpaired t test was used for variables that fit within the normal distribution. In addition, the association between the initial RV dimensions quantified by CT and the type of final surgery performed was evaluated in the hypoplastic RV group. Statistical analyses were performed using the IBM SPSS Statistics for Windows, Version 24.0 (IBM Corp., Armonk, NY, USA). A p value < 0.05 was considered statistically significant.
In the hypoplastic RV group, we found that both age and body surface area were significantly smaller than those measured in the control group (p = 0.012 and 0.016, respectively) (Table 1).
The RV and LV volume and length were successfully quantified from the cardiothoracic CT data in all 90 patients. However, the tricuspid valve annulus diameters could not be measured in 44.64% (25/56) of the cases in the ES phase, and in 23.81% (5/21) of the cases in the ED phase. These cases involved patients with tricuspid atresia with a muscular partition (Fig. 1) and double-inlet LV. As a result, the AVV annulus diameter ratio could not be calculated for these patients even though the mitral valve annulus diameter was measured. The AVV annulus diameter ratio was successfully calculated in the remaining patients, including 5 cases of a rare type of tricuspid atresia with imperforate valve (Fig. 2).
In both the ES and ED cardiac phases, the indexed RV volumes, the RV/LV volume ratio, the AVV annulus diameter ratio, and the RV/LV length ratio were significantly lower in the hypoplastic RV group than in the control group (p < 0.001) (Table 1).
In the hypoplastic RV group, the types of final surgery performed were univentricular repair procedures such as Fontan operation or classical or pulsatile bidirectional cavopulmonary connection in 46 patients and biventricular repair procedures in 4 patients, while the surgical procedure was classified as indeterminate in 7 patients. Notably, biventricular repair was conducted in 2 patients with unbalanced atrioventricular septal defects and trisomy 21 and 2 patients with pulmonary atresia and an intact ventricular septum. Thus, all patients with unbalanced atrioventricular septal defects included in this study underwent biventricular repair using a two-patch technique, with one patient dying following the biventricular repair. The RV indexed end-systolic volume (15.9 and 22.5 mL/m2), RV indexed end-diastolic volume (44.5 and 59.0 mL/m2), ES RV/LV volume ratio (0.56, 0.68), and ED RV/LV volume ratio (0.64, 0.69) in the 2 patients with unbalanced atrioventricular defects were greater than 2 standard deviations above the mean, whereas the values in the 2 patients with pulmonary atresia with an intact ventricular septum were not. In contrast, the AVV annulus diameter ratio and the RV/LV length ratio were comparable between these 2 cardiac defects that were corrected by biventricular repair. The 7 indeterminate surgery cases were so categorized due to either unavailable follow-up data in 4 patients or death in 3 of patients. The total clinical follow-up period ranged from 3 to 8 years.
This study demonstrated that, although the tricuspid valve annulus diameter was not measurable in conditions such as muscular tricuspid atresia and double-inlet LV, all the other initial RV dimensions, including ventricular volume, mitral valve annulus diameter, and ventricular length, could be quantified using CT in infants with CHD and a hypoplastic RV. The CT-quantified RV dimensions were significantly smaller in the hypoplastic RV group than in the control group. These results suggest that cardiothoracic CT may be used effectively for evaluating a hypoplastic RV objectively and noninvasively in patients with CHD.
In this study, the mean ES and ED RV volumes in the hypoplastic RV group were respectively 54.74% (= 9.87 mL/m2 / 18.03 mL/m2 × 100) and 37.59% (= 20.60 mL/m2 / 54.80 mL/m2 × 100) of the corresponding volumes in the control group. In a previous study (1), the mean RV volume measured by echocardiography in 5 patients with a hypoplastic RV was 48.4% of the predicted value, which is comparable to the results in this study. In comparison with the mean RV/LV volume ratios in the hypoplastic RV group that ranged from 0.25 to 0.30 in this study, the RV/LV volume ratios measured in 8 patients with a hypoplastic RV by echocardiography in a previous study were relatively low, ranging from 0.09 to 0.25 (2). This discrepancy may be attributed to underestimation of the echocardiographic RV volumes, which is regarded as one of the shortcomings of echocardiography, even when using a 3D acquisition technology (19).
The AVV index obtained using the area or diameter ratio and the RV/LV length ratio measured by echocardiography are commonly used for evaluating the size of the RV (34620). In a previous study with 38 patients with unbalanced atrioventricular septal defects and a small RV, the AVV index was 0.64 ± 0.26 and the RV/LV length ratio was 0.80 ± 0.10 for the biventricular repair group (n = 32) and 0.65 ± 0.08 and 0.62 ± 0.13 for the univentricular repair group (n = 6) (3). The small sample size of the biventricular repair group in the previous study (3), however, precluded a meaningful statistical analysis. A different study (6) demonstrated that an AVV index > 0.79 was a good predictor of biventricular repair, with a specificity of 100% and a positive predictive value of 100% in 36 patients with pulmonary atresia, intact ventricular septum, and a hypoplastic RV. Although several types of cardiac defects were included here, the mean AVV annulus diameter ratios ranging from 0.59 to 0.63 were comparable to the published results (3) and the mean RV/LV length ratios ranging from 0.60 to 0.61 were similar to those for the univentricular repair group reported in other studies (36). In line with these findings, the majority of patients in the hypoplastic RV group (80.70%, 46/57) underwent a univentricular repair.
Because trisomy 21 is commonly associated with a left-dominant unbalanced atrioventricular septal defect and these patients frequently have upper airway obstruction and early increased pulmonary vascular resistance, a univentricular repair with Fontan physiology is challenging in these circumstances and a more aggressive treatment using a biventricular repair instead tends to be adopted in these patients (3). This occurred in our study as well, where biventricular repair was performed in two patients with left-dominant unbalanced atrioventricular septal defect and trisomy 21. Successful biventricular repair using leftward partitioning of the AVV to expand the RV inflow tract was reported in an infant with left-dominant unbalanced atrioventricular septal defect and trisomy 21, although the AVV index (0.42) and the RV/LV length ratio (0.67) measured by echocardiography were marginally small to predict a successful biventricular repair (20).
In 40 patients with pulmonary atresia and an intact ventricular septum, a disproportional growth of the tricuspid valve compared to somatic growth was observed after decompression of the RV, particularly for patients presenting with small tricuspid valves and lower RV pressures after decompression (21). The CT-quantified RV dimensions used in the present study may be useful for monitoring this growth potential in such patients.
A major limitation of this study was that it involved a retrospective study design and a heterogeneous study population. However, cardiac defects with a hypoplastic RV such as left-dominant unbalanced atrioventricular septal defect are extremely rare. Although harder to develop, a prospective study including more homogeneous cardiac defects may allow for clinically meaningful results in evaluating a hypoplastic RV. The main objective of this study was to investigate the feasibility of CT quantification of the initial RV dimensions in infants with CHD and a hypoplastic RV, which had not been utilized before. Because the gold standard for RV size is difficult to achieve in clinical studies such as this one, a control group was used for comparison. However, the age and body surface area of the control group were not perfectly matched to those in the hypoplastic RV group. The small sample size (n = 4) of the biventricular group prevented statistical analysis for the prediction of the type of final surgery based on the CT-quantified initial RV dimensions. Paired ES and ED phases could not be obtained in the hypoplastic RV group as a result of the retrospective study design, in which cardiothoracic CT was performed to answer different clinical questions in the hypoplastic RV group.
In conclusion, initial RV dimensions in infants with CHD and a hypoplastic RV can be quantified by CT and were substantially smaller than those in infants with tetralogy of Fallot. Among the dimensions measured, it was not possible to obtain the AVV annulus diameter ratio in cases presenting with muscular tricuspid atresia or double-inlet LV. Cardiothoracic CT appears to be useful to obtain the initial RV dimensions, particularly in patients with complex CHD and a borderline small RV, thereby facilitating decisions regarding optimal treatment strategies.
References
1. Muster AJ, Zales VR, Ilbawi MN, Backer CL, Duffy CE, Mavroudis C. Biventricular repair of hypoplastic right ventricle assisted by pulsatile bidirectional cavopulmonary anastomosis. J Thorac Cardiovasc Surg. 1993; 105:112–119. PMID: 8419691.


2. Gentles TL, Keane JF, Jonas RA, Marx GE, Mayer JE Jr. Surgical alternatives to the Fontan procedure incorporating a hypoplastic right ventricle. Circulation. 1994; 90(5 Pt 2):II1–II6.
3. De Oliveira NC, Sittiwangkul R, McCrindle BW, Dipchand A, Yun TJ, Coles JG, et al. Biventricular repair in children with atrioventricular septal defects and a small right ventricle: anatomic and surgical considerations. J Thorac Cardiovasc Surg. 2005; 130:250–257. PMID: 16077383.
4. Yoshimura N, Yamaguchi M. Surgical strategy for pulmonary atresia with intact ventricular septum: initial management and definitive surgery. Gen Thorac Cardiovasc Surg. 2009; 57:338–346. PMID: 19597923.


5. Awori MN, Mehta NP, Mitema FO, Kebba N. Optimal Z-score use in surgical decision-making in pulmonary atresia with intact ventricular septum. World J Pediatr Congenit Heart Surg. 2017; 8:385–388. PMID: 28520535.


6. Chen RHS, Chau AKT, Chow PC, Yung TC, Cheung YF, Lun KS. Achieving biventricular circulation in patients with moderate hypoplastic right ventricle in pulmonary atresia intact ventricular septum after transcatheter pulmonary valve perforation. Congenit Heart Dis. 2018; 13:884–891. PMID: 30238621.


7. Goo HW, Park SH. Semiautomatic three-dimensional CT ventricular volumetry in patients with congenital heart disease: agreement between two methods with different user interaction. Int J Cardiovasc Imaging. 2015; 31 Suppl 2:223–232. PMID: 26319216.


8. Kim HJ, Goo HW, Park SH, Yun TJ. Left ventricle volume measured by cardiac CT in an infant with a small left ventricle: a new and accurate method in determining uni- or biventricular repair. Pediatr Radiol. 2013; 43:243–246. PMID: 22875206.


9. Goo HW. Serial changes in anatomy and ventricular function on dual-source cardiac computed tomography after the Norwood procedure for hypoplastic left heart syndrome. Pediatr Radiol. 2017; 47:1776–1786. PMID: 28879411.


10. Goo HW, Park SH. Computed tomography-based ventricular volumes and morphometric parameters for deciding the treatment strategy in children with a hypoplastic left ventricle: preliminary results. Korean J Radiol. 2018; 19:1042–1052. PMID: 30386136.


11. Goo HW, Suh DS. Tube current reduction in pediatric non-ECG-gated heart CT by combined tube current modulation. Pediatr Radiol. 2006; 36:344–351. PMID: 16501970.


12. Goo HW. State-of-the-art CT imaging techniques for congenital heart disease. Korean J Radiol. 2010; 11:4–18. PMID: 20046490.


13. Goo HW. Individualized volume CT dose index determined by cross-sectional area and mean density of the body to achieve uniform image noise of contrast-enhanced pediatric chest CT obtained at variable kV levels and with combined tube current modulation. Pediatr Radiol. 2011; 41:839–847. PMID: 21656275.


14. Goo HW. Is it better to enter a volume CT dose index value before or after scan range adjustment for radiation dose optimization of pediatric cardiothoracic CT with tube current modulation? Korean J Radiol. 2018; 19:692–703. PMID: 29962875.


15. Hong SH, Goo HW, Maeda E, Choo KS, Tsai IC;. User-friendly vendor-specific guideline for pediatric cardiothoracic computed tomography provided by the Asian Society of Cardiovascular Imaging Congenital Heart Disease Study Group: part 1. Imaging techniques. Korean J Radiol. 2019; 20:190–204. PMID: 30672159.


16. Tricarico F, Hlavacek AM, Schoepf UJ, Ebersberger U, Nance JW Jr, Vliegenthart R, et al. Cardiovascular CT angiography in neonates and children: image quality and potential for radiation dose reduction with iterative image reconstruction techniques. Eur Radiol. 2013; 23:1306–1315. PMID: 23207869.


17. Goo HW. Combined prospectively electrocardiography- and respiratory-triggered sequential cardiac computed tomography in free-breathing children: success rate and image quality. Pediatr Radiol. 2018; 48:923–931. PMID: 29589058.


18. Goo HW. Semiautomatic three-dimensional threshold-based cardiac computed tomography ventricular volumetry in repaired tetralogy of Fallot: comparison with cardiac magnetic resonance imaging. Korean J Radiol. 2019; 20:102–113. PMID: 30627026.


19. Shiota T. 3D echocardiography: evaluation of the right ventricle. Curr Opin Cardiol. 2009; 24:410–414. PMID: 19550311.


20. Oshima Y, Maruo A, Hasegawa T, Matsuhisa H. Repair of unbalanced atrioventricular septal defect with small right ventricle. Asian Cardiovasc Thorac Ann. 2016; 24:78–80. PMID: 25061220.


21. Huang SC, Ishino K, Kasahara S, Yoshizumi K, Kotani Y, Sano S. The potential of disproportionate growth of tricuspid valve after decompression of the right ventricle in patients with pulmonary atresia and intact ventricular septa. J Thorac Cardiovasc Surg. 2009; 138:1160–1166. PMID: 19837219.


Fig. 1
Initial cardiothoracic CT in 8-day-old female newborn with muscular tricuspid atresia.
A. Four-chamber image shows muscular atresia (arrows) of tricuspid valve and hypoplastic RV. Mitral valve annulus diameter (black line) and length of RV and LV (gray lines) were measurable. Tricuspid valve annulus diameter was not measurable. As result, atrioventricular valve annulus diameter ratio could not be calculated. B. RV ESVi quantified with CT ventricular volumetry was 4.7 mL/m2. C. LV ESVi quantified with CT ventricular volumetry was 30.0 mL/m2.
CT = computed tomography, ESVi = indexed end-systolic volume, LA = left atrium, LV = left ventricle, RA = right atrium, RV = right ventricle
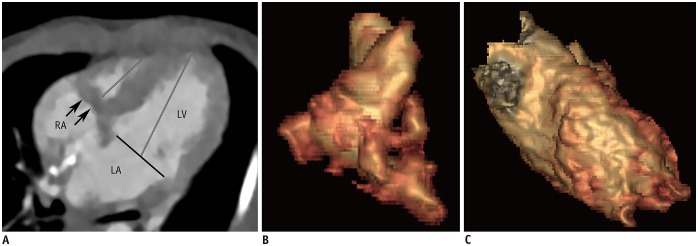
Fig. 2
Initial cardiothoracic CT in 4-day-old female newborn with tricuspid atresia with imperforate valve.
A. Four-chamber image shows imperforated tricuspid valve (arrow) and hypoplastic RV. In contrast to muscular type of tricuspid atresia, annulus diameters (black lines) of tricuspid and mitral valves as well as length of RV and LV (gray lines) were measurable. B. RV ESVi quantified with CT ventricular volumetry was 6.2 mL/m2. C. LV ESVi quantified with CT ventricular volumetry was 39.0 mL/m2.
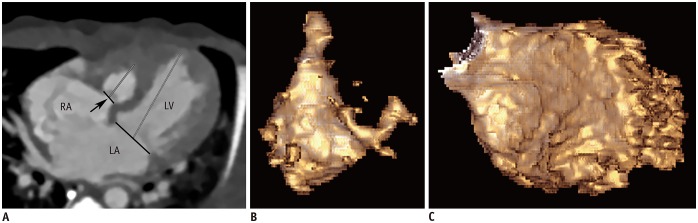
Table 1
Comparison of Age, BSA, and CT-quantified RV Dimensions and Ratios between Hypoplastic RV and Control Groups
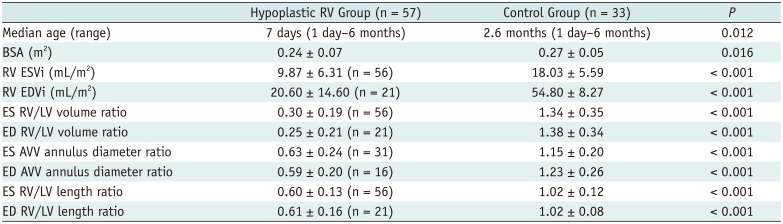