Abstract
Purpose
Materials and Methods
Results
Figures and Tables
Fig. 1
Long non-coding RNA anti-differentiation noncoding RNA (lncRNA-ANCR) expression analysis based on a mouse postmenopausal osteoporosis (PMOP) model. The PMOP model was constructed using three groups: osteoporosis group, control group, and sham group (n=15 each group). (A) Bone mineral density comparison using microcomputed tomography. (B) Laser confocal microscopy detection for calcium level (×100, Fluo8 fluorescent probes are labeled). (C) Quantitative real-time polymerase chain reaction (qRT-PCR) analysis for lncRNA-ANCR mRNA and protein expression in osteoblast cells of the PMOP model. X-axis in C represents lncRNA-ANCR relative mRNA expression levels; Y-axis presents different groups in the PMOP model. *p<0.001 vs. Control group and Sham group.
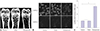
Fig. 2
mRNA expression of long non-coding RNA anti-differentiation noncoding RNA (lncRNA-ANCR) in osteoblast cells transfected with siRNA-ANCR (si-ANCR). X-axis presents relative lncRNA-ANCR expression; Y-axis presents the three groups in postmenopausal osteoporosis model. *p<0.001 vs. NC-ANCR (negative control group, cell line was transfected with lncRNA-ANCR negative control) group and BLANK group (blank control group, cells without treatment).
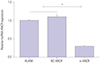
Fig. 3
Effects of long non-coding RNA anti-differentiation noncoding RNA (lncRNA-ANCR) transfected with siRNA-ANCR (si-ANCR). (A) The effect of lncRNA-ANCR on proliferation evaluated by MTT assay. (B) The effect of lncRNA-ANCR on apoptosis determined by flow cytometry. (C) The effect of lncRNA-ANCR on alkaline phosphatase (ALP) activity detected by ALP staining. X-axis represents the relative lncRNA-ANCR expression; Y-axis presents the three groups in the postmenopausal osteoporosis model. (D) The effect of lncRNA-ANCR on calcium deposition upon alizarin red staining. *p<0.05, †p<0.01, ‡p<0.001 vs. NC-ANCR group (negative control group, cell line was transfected with lncRNA-ANCR negative control) and BLANK group (blank control group, cells without treatment). PI, propidium iodide; FITC, fluoresceine isothiocyanate; OD, optical density.
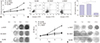
Fig. 4
In vivo experiments show the regulatory effect of long non-coding RNA anti-differentiation noncoding RNA (lncRNA-ANCR) on osteoid formation. (A) Hematoxylin-eosin (HE) staining of osteoid formation in mice injected with siRNA-ANCR (si-ANCR)-transfected osteoblast cells (bar=200 µm). Black and gray arrows indicate hydroxyapatite-tricalcium phosphate and osteoblast cells, respectively. (B) Osteoid/Total area (%). *p<0.01 vs. NC-ANCR group (negative control group, cell line was transfected with lncRNA-ANCR negative control) and BLANK group (blank control group, cells without treatment).
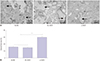
Fig. 5
Expression of enhancer of zeste homolog 2 (EZH2), runt related transcription factor 2 (RUNX2), and OSTERIX in osteoblast cells transfected with siRNA-anti-differentiation noncoding RNA (si-ANCR). (A) Quantitative real-time polymerase chain reaction (qRT-PCR) results for the mRNA and protein expression of EZH2, Runx2, and Osterix. (B and C) Western blot results for mRNA and protein expression of EZH2, RUNX2, and OSTERIX. *p<0.001 vs. NC-ANCR group (negative control group, cell line was transfected with lncRNA-ANCR negative control) and BLANK group (blank control group, cells without treatment). GAPDH, glyceraldehyde-3-phosphate dehydrogenase.
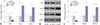
Fig. 6
The interaction between long non-coding RNA anti-differentiation noncoding RNA (lncRNA-ANCR) and enhancer of zeste homolog 2 (EZH2). (A) Expression of EZH2 in ANCR and ANCR-Mut detected by quantitative real-time polymerase chain reaction (qRT-PCR) and Western blot. (B) The expression of ANCR in EZH2 and EZH2-control detected by qRT-PCR and Western blot. *p<0.001, lncRNA-ANCR group vs. lncRNA-ANCR-Mut group, EZH2 group vs. EZH2-control group.
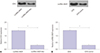
ACKNOWLEDGEMENTS
Notes
AUTHOR CONTRIBUTIONS
Conceptualization: Nuoya Cai.
Data curation: Nuoya Cai.
Formal analysis: Nuoya Cai.
Funding acquisition: Chao Li.
Investigation: Chao Li.
Methodology: Chao Li.
Project administration: Nuoya Cai.
Resources: Nuoya Cai.
Software: Chao Li.
Supervision: Chao Li.
Validation: Fuke Wang.
Visualization: Fuke Wang.
Writing—original draft: Nuoya Cai, Chao Li, and Fuke Wang.
Writing—review & editing: Nuoya Cai, Chao Li, and Fuke Wang.
References






























