Abbreviations
APC
ATRA
BM
CD11chi
CTV
DCs
F4/80hi
FCS
FITC-OVA
FLT3L
FOXP3
GEO
GSE
ICAM2
i.p.
IRF4
iTreg
KO
Lin
LPM
MHCIIlo
MoDC
NOTCH2
OVA
RELMα
RNA-Seq
SPM
Tc
YGM
INTRODUCTION
MATERIALS AND METHODS
Animals
Abs and reagents
Flow cytometry
Ag uptake
Ag presentation and T cell proliferation
Cytokine-induced cell expansion in vivo
RNA sequencing (RNA-Seq) and bioinformatics analysis
Statistical analysis
RESULTS
Phenotypic characterization of peritoneal myeloid mononuclear cells
Figure 1
Classification of peritoneal myeloid mononuclear cells. Cells in the peritoneal cavity are harvested and analyzed by multi-parameter flow cytometry. (A) The gating strategies to divide peritoneal myeloid mononuclear cells. Cells expressing Lin markers of CD3, CD19, and Ly6G are excluded. (B) Distinct expression of myeloid cell markers. P-IV subset is further divided into P-IV.I and P-IV.II based on the expression levels of CD14 and CD206 (in red box). (C) The number of cells in each subset is evaluated from the peritoneal cavity. Data are shown with SEM from at least 5 independent experiments. Each experiment analyzed peritoneal exudate cells from a single mouse.
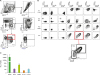
Characterization of morphology and Ag uptake
Figure 2
Morphology and Ag uptake ability are examined for each subset of peritoneal myeloid mononuclear cells. (A) Peritoneal exudate cells are harvested and sorted by flow cytometry according to the gates as in Fig. 1. Isolated cells in each subset are cultured overnight before taking pictures under 200× magnification. Cells in each subset are compared for their ability to take up Ags via endocytosis (B) or phagocytosis (C). (B) 200 μg of soluble FITC-conjugated OVA proteins are injected i.p. After 1 h, peritoneal exudate cells are harvested and analyzed. The extents of Ag uptake are assessed by the geometric mean fluorescence intensity index of the FITC channel. Each gray-filled histogram is from each subset in control uninjected mice. Overlay of histograms on FITC channel from each subset is shown on the right lower side. Representative data shown are from 2 independent experiments. (C) A volume of 200 μl of 0.0027% YGM (1.0 μm diameter) is injected i.p. After 1 h, peritoneal exudate cells are harvested and analyzed. Percentages of cells that phagocytose more than 1, 2, or 3 beads are denoted in order. Overlay of histograms on YG channel from each subset is shown on the right lower side. Representative data shown are from 2 independent experiments.
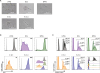
Comparison of Ag-presenting ability to stimulate naïve T cells
Figure 3
Comparison of Ag-presenting ability to stimulate naïve T cells. (A, B) 1.5 mg of OVA Ag are injected i.p. After 1 h, peritoneal exudate cells are harvested and sorted by flow cytometry according to the gates as in Fig. 1. Isolated cells in each subset are cultured with 25,000 CTV-labeled OT-1 or OT-2 T cells in the indicated APC:TC ratio. The numbers and percentages of CTVlo proliferated OT-1 and OT-2 T cells are analyzed on day 3 and day 4 respectively. Representative flow cytometric plots of OT-1 (left panels) and OT-2 (right panels) TCs are shown. Data are shown from more than 2 independent experiments. Error bars indicate mean±SEM across multiplicate sample.
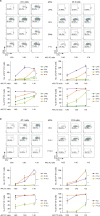
Distinct differentiation of T cells by peritoneal APCs
Figure 4
Distinct differentiation of T cells by peritoneal APCs. As in Fig. 3, from mice treated with OVA Ag, peritoneal APCs, i.e., DCs and P-IV.I cells, are sorted by flow cytometry. Isolated 2,500 cells of respective APCs are co-cultured with 25,000 OT-1 or OT-2 T cells for 3 or 4 days respectively. (A) Proliferated T cells are stained and analyzed for specific markers, CD25, CD69, CD44, and CD62L. Cells with indicated phenotypes (i.e., CD25+, CD69+, CD44+, and CD62L−) and their respective percentages among the proliferated (i.e., CTVlo) T cells are gated and denoted in representative flow cytograms. Bar graphs are drawn from triplicate samples. (B, C) PMA, ionomycin and brefeldin A are treated for the last 4 h of culture. Indicated cytokines are stained intracellularly. Represented flow cytometric plots of live CTVlo CD8+ OT-1 (B) or CD4+ OT-2 (C) T cells are shown. Bar graphs illustrate the frequencies of described cytokine producing OT-1 (B) and OT-2 (C) T cells among total proliferated T cells. (D) hTGF-β and/or ATRA are added to the wells containing OVA-laden APCs and OT-2 T cells. Foxp3 is stained intracellularly followed by the staining and fixation of surface molecules. Live CTVlo CD4+ T cells are shown, and iTreg cells are gated and denoted with frequencies. Representative data are shown from at least 2 independent experiments. Error bars indicate mean±SEM across multiplicate sample.
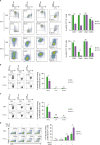
Influence of hematopoietic cytokines on the development of subpopulations
Figure 5
Influence of hematopoietic cytokines on the development of peritoneal myeloid mononuclear subsets. Mice are injected subcutaneously with a hematopoietic cytokine daily for 3 (for GM-CSF) to 5 (for FLT3L and M-CSF) consecutive days. (A) Representative flow cytometric plots of peritoneal myeloid mononuclear subsets are shown from the mice treated with each hematopoietic cytokine. Numbers indicate frequencies of gated cells in each population. Data in graph are calculated from at least 3 mice, and each dot represents a mouse. (B) Numbers of cells in peritoneal myeloid mononuclear subsets are detected from FLT3−/− and GM-CSF−/− mice. Data in graph are calculated from at least 3 mice, and each dot represents a mouse. Error bars indicate mean±SEM across multiplicate sample.
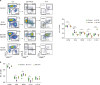
Gene expression profiles of MHCII+ peritoneal myeloid mononuclear cells
Figure 6
Comparison of gene expression profiles of MHCII+ peritoneal myeloid mononuclear cell subsets. (A) The gating strategy to categorize peritoneal DC subsets. Peritoneal DCs, gated as in Fig. 1A, are further divided into DC1 and DC2 subsets by the surface expression levels of XCR1 and CD11b. RNA-Seq is performed with mRNA samples from the respective subsets of MHCII+ peritoneal myeloid mononuclear cells isolated by flow cytometric sorting on the gating strategies as in Figs. 1A and 6A. (B) Multidimensional scaling analysis of principal components from RNA-Seq profiles of MHCII+ peritoneal myeloid mononuclear cell subsets. (C-F) Heat maps show the genes differentially expressed among MHCII+ peritoneal myeloid mononuclear cell subsets. Heat maps for the differential expression of genes related to macrophages (C), cDCs (D), DC-associated transcription factors (E), and monocytes/MoDCs (F) are shown. The color scale represents the row Z score.
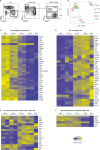