INTRODUCTION
MATERIALS AND METHODS
Cell culture
The SMF exposure system
![]() | Figure 1Effects of SMFs on osteoblastic differentiation in human osteoblasts. (A) Schematic diagram of the SMF exposure system. (B) Cell proliferation was examined by an MTT assay at 3, 7, and 14 days. (C) Differentiation was assessed based on ALP activity, (D) Alizarin red staining, (E) calcium content, and (F) expression of the mRNA of bone matrix proteins. The intensity of Alizarin red staining was determined by optical density. Cells were treated with OS medium containing 50 μg/mL of L-ascorbic acid and 10 mM β-glycerophosphate along with 3-, 15-, and 50-mT SMFs for 7 days and 14 days. OM contained OS and 10−7 M dexamethasone. The results are representative of 5 independent experiments that were performed.
SMF: static magnetic field, MTT: 3-(4,5-dimethylthiazol-2-yl)-5-(3-carboxymethoxyphenyl)-2-(4-sulfophenyl)-2H-tetrazolium, ALP: alkaline phosphatase, OS: osteogenic supplement, OM: osteogenic medium, Runx2: runt-related transcription factor 2, OPN: osteopontin, OCN: osteocalcin, GAPDH: glyceraldehyde 3-phosphate dehydrogenase.
a)Significant difference compared to control (P<0.05); b)Significant difference between each group (P<0.05).
|
Cell viability
Alkaline phosphatase (ALP) activity
Alizarin red staining
RNA isolation and reverse transcription polymerase chain reaction (RT-PCR)
Table 1
RT-PCR primers and conditions
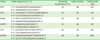
Western blot analysis
Ca2+ assays
Immunocytochemistry
Statistical analysis
RESULTS
SMFs promote osteoblastic differentiation in human osteoblasts
SMFs promote osteoblastic/cementoblastic differentiation in PDLCs and cementoblasts
![]() | Figure 2Effect of SMFs on osteoblastic differentiation in a cell line of human PDLCs and cementoblasts. (A, B) Cell proliferation was examined by an MTT assay at 3, 7, and 14 days. (C, D) ALP activity was determined and normalized to protein content. (E, F) Matrix mineralization was evaluated by Alizarin red staining. Cells were treated with 15-mT SMFs and OS or OM for 14 days. The results are representative of 5 independent experiments.
SMF: static magnetic field, PDLC: periodontal ligament cell, MTT: 3-(4,5-dimethylthiazol-2-yl)-5-(3-carboxymethoxyphenyl)-2-(4-sulfophenyl)-2H-tetrazolium, ALP: alkaline phosphatase, OS: osteogenic supplement, OM: osteogenic medium.
a)Significant difference compared to control (P<0.05); b)Significant difference between each group (P<0.05).
|
![]() | Figure 3Effects of SMFs on calcium content and osteoblastic or cementoblastic gene expression in a cell line of human PDLCs and cementoblasts. (A, B) Ca2+ concentration was measured using a calcium quantification assay kit. (C, D) mRNA was determined by RT-PCR analysis. Cells were treated with a 15-mT SMF and OS or OM for 14 days. The results are representative of 5 independent experiments.
SMF: static magnetic field, PDL: periodontal ligament, PDLC: periodontal ligament cell, RT-PCR: reverse transcription polymerase chain reaction, OS: osteogenic supplement, OM: osteogenic medium, Runx2: runt-related transcription factor 2, OPN: osteopontin, OCN: osteocalcin, GAPDH: glyceraldehyde 3-phosphate dehydrogenase, CEMP-1: cementum protein 1, CAP: cementum-derived attachment protein.
a)Significant difference compared to control (P<0.05); b)Significant difference between each group (P<0.05).
|
SMFs promote osteoblastic differentiation in primary cultured osteoblasts and PDLCs
![]() | Figure 4Effect of SMFs on proliferation, ALP activity, and mineralized nodule formation in primary cultured human PDLCs and osteoblasts. (A, B) Cell proliferation was examined by an MTT assay at 3, 7, and 14 days. (C, D) ALP activity was determined and normalized to protein content. (E, F) Matrix mineralization was evaluated by Alizarin red staining as described in the Materials and Methods. Cells were treated with 15-mT SMFs and OS or OM for 14 days. The results are representative of 5 independent experiments.
SMF: static magnetic field, ALP: alkaline phosphatase, PDLC: periodontal ligament cell, MTT: 3-(4,5-dimethylthiazol-2-yl)-5-(3-carboxymethoxyphenyl)-2-(4-sulfophenyl)-2H-tetrazolium, OS: osteogenic supplement, OM: osteogenic medium.
a)Significant difference compared to control (P<0.05); b)Significant difference between each group (P<0.05).
|
![]() | Figure 5Effect of SMFs on calcium content and osteoblastic gene expression in primary cultured human PDLCs and osteoblasts. (A, B) Ca2+ concentration was measured using a calcium quantification assay kit. (C, D) mRNA was determined by RT-PCR analysis. The results are representative of 5 independent experiments.
SMF: static magnetic field, PDLC: periodontal ligament cell, RT-PCR: reverse transcription polymerase chain reaction, Runx2: runt-related transcription factor 2, OPN: osteopontin, OCN: osteocalcin, GAPDH: glyceraldehyde 3-phosphate dehydrogenase.
a)Significant difference compared to control (P<0.05); b)Significant difference between each group (P<0.05).
|
SMFs activate Wnt/β-catenin, p38 and c-Jun N-terminal kinase (JNK) mitogen-activated protein kinases (MAPKs), and nuclear factor-κB (NF-κB) signaling, but not extracellular signal-regulated kinase (ERK) signaling
![]() | Figure 6Effects of SMFs on the Wnt/β-catenin, MAPK, and NF-κB signaling pathways in human osteoblasts. (A, B) Cells were treated with SMFs and OS or OM for 2 days, (C) 30 minutes, and (D, E) 45 minutes. (A-D) Protein levels were assessed by western blot analysis and (E) immunofluorescence staining. Arrows (yellow) indicate the nuclear translocation of NF-κB p65. The data presented are representative of five independent experiments.
SMF: static magnetic field, MAPK: mitogen-activated protein kinase, NF-κB: nuclear factor-κB, OS: osteogenic supplement, OM: osteogenic medium, GSK-3β: glycogen synthase kinase-3β, ERK: extracellular signal-regulated kinase, JNK: c-Jun N-terminal kinase.
|
![]() | Figure 7Effects of various inhibitors of signal transduction on SMF-induced osteoblastic differentiation in human osteoblasts. (A-D) Cells were pretreated for 2 hours with DKK1 (0.5 μg/mL), SB203580 (20 μM), PD98059 (20 μM), SP600125 (10 μM), and PDTC (10 μM), and then cultured in OS with 15-mT SMFs for 14 days. The results are representative of 5 independent experiments.
SMF: static magnetic field, OS: osteogenic supplement, Runx2: runt-related transcription factor 2, OPN: osteopontin, OCN: osteocalcin, GAPDH: glyceraldehyde 3-phosphate dehydrogenase.
a)Significant difference between each group (P<0.05).
|
DISCUSSION
![]() | Figure 8Schematic diagram illustrating the Wnt, Akt, MAPK, and NF-κB signaling pathways triggered by exposure to SMFs, which ultimately stimulate the osteoblastic differentiation of human osteoblasts.
MAPK: mitogen-activated protein kinase, NF-κB: nuclear factor-κB, SMF: static magnetic field, Fzd: Frizzled, GSK-3β: glycogen synthase kinase-3β, JNK: c-Jun N-terminal kinase.
|