Abstract
Purpose
Alzheimer's disease (AD) is the sixth most common cause of death in the United States. MicroRNAs have been identified as vital players in neurodegenerative diseases, including AD. microRNA-128 (miR-128) has been shown to be dysregulated in AD. This study aimed to explore the roles and molecular mechanisms of miR-128 in AD progression.
Materials and Methods
Expression patterns of miR-128 and peroxisome proliferator-activated receptor gamma (PPAR-γ) messenger RNA in clinical samples and cells were measured using RT-qPCR assay. PPAR-γ protein levels were determined by Western blot assay. Cell viability was determined by MTT assay. Cell apoptotic rate was detected by flow cytometry via double-staining of Annexin V-FITC/PI. Caspase 3 and NF-κB activity was determined by a Caspase 3 Activity Assay Kit or NF-κB p65 Transcription Factor Assay Kit, respectively. Bioinformatics prediction and luciferase reporter assay were used to investigate interactions between miR-128 and PPAR-γ 3′UTR.
Results
MiR-128 expression was upregulated and PPAR-γ expression was downregulated in plasma from AD patients and amyloid-β (Aβ)-treated primary mouse cortical neurons (MCN) and Neuro2a (N2a) cells. Inhibition of miR-128 decreased Aβ-mediated cytotoxicity through inactivation of NF-κB in MCN and N2a cells. Moreover, PPAR-γ was a target of miR-128. PPAR-γ upregulation attenuated Aβ-mediated cytotoxicity by inactivating NF-κB in MCN and N2a cells. Furthermore, PPAR-γ downregulation was able to abolish the effect of anti-miR-128 on cytotoxicity and NF-κB activity in MCN and N2a cells.
Alzheimer's disease (AD), a neurodegenerative disorder, is the sixth most common cause of death in the United States, with an increase of 71% in AD-induced deaths between 2000 and 2013.1 It is estimated that there will be one million new cases per year and a total estimated prevalence of 13.8 million by 2050 in the United States.1 AD is characterized by intracellular neurofibrillary tangles (NFTs) formation and extracellular amyloid-β (Aβ) deposition, together with a loss or impairment of language, memory, behavior, and cognition abilities.2 NFTs are composed of insoluble and hyperphosphorylated microtubule-binding protein tau, and Aβ is produced from cleavage of amyloid precursor protein (APP).2 Although much progress has been made in the diagnosis and management of AD over the past decades, currently, therapeutic agents are only able to retard AD progression with limited efficiency.34 Hence, deeper insight into the molecular basis of AD may help to identify some effectively therapeutic strategies.
Recently, an amyloid hypothesis proposes that Aβ accumulation contributes to the initiation of AD by causing hyperphosphorylation of tau and damage to neurons and synapses.56 In nervous systems, NF-κB has been identified as a critical regulator in multiple biological processes, such as neuronal survival, inflammation, and apoptosis.7 Moreover, exogenous increases in Aβ can activate NF-κB signaling in both neuronal cells and microglial cells, and NF-κB is implicated in AD pathogenesis by regulating upstream regulators and downstream effectors.8
MicroRNAs (miRNAs), a class of small noncoding ribonucleic acids with the length about 18–25 nucleotides (nt), regulate messenger RNA (mRNA) activity at posttranscriptional levels.9 MiRNAs have been identified as vital players in neurodegenerative diseases, such as AD, Huntington's disease, or Parkinson's disease.10 A prior study pointed out that microRNA-128 (miR-128), miR-9, miR-7, miR-125, miR-23b, miR-132, miR-137, and miR-139 are substantially enriched in brains.11 Also, data analysis of the GEO database (GSE36981, expression data from Alzheimer's disease model mouse) showed that miR-128 expression is notably altered, compared with other miRNAs (miR-9, miR-7, miR-125, miR-23b, miR-132, miR-137, and miR-139), hinting at roles for miR-128 in the development of AD. Moreover, miR-128 has been demonstrated to be highly expressed in the hippocampus of AD patients relative to age-matched controls.12 Müller, et al.13 further demonstrated that miR-128 expression is upregulated in the hippocampus in an intermediate stage of AD patients and to be downregulated in the late stage of AD patients. These data suggested that miR-128 might play a role in AD pathogenicity. Hence, the roles and molecular mechanisms of miR-128 in AD progression have been further explored.
Peroxisome proliferator-activated receptor gamma (PPAR-γ), a ligand-activated transcription factor, has been implicated in the pathogenesis of AD.14 Moreover, decreased PPAR-γ expression has been demonstrated in AD brain.15 Additionally, previous studies showed that activation of PPAR-γ could inhibit Aβ-induced inflammation and neurotoxicity.1617
In the present study, we demonstrated that miR-128 exerts its cytotoxic effect by targeting PPAR-γ via regulation of the NF-κB pathway in Aβ-stimulated mouse neuron cells.
Our study was approved by the Ethic Committee of Huaihe Hospital of Henan University, and signed consent was obtained from every participant. Blood samples (10 mL) were collected from AD patients (n=20) and age and education-matched healthy volunteers (n=20) at our hospital in EDTA vacutainers, followed by isolation of plasma by centrifugation (3000 rpm, 5 min). AD patients (70±5 years old, male:female=2:3) were diagnosed following the International Working Group (IWG)-2 criteria for Alzheimer's disease.18 The severity of dementia was evaluated using an updated standard of the Clinical Dementia Rating scale (CDR)19 and Mini-Mental Status Examination (MMSE).20 All AD patients were in CDR2 stage and exhibited moderate cognitive impairment with mean MMSE scores of about 15. Healthy people (70±5 years old; male:female=2:3) with MMSE scores higher than 28 were recruited following the standards without any cognitive impairment, dementia, and other disease.
Mouse neuroblast Neuro2a (N2a) cells were purchased from American Type Culture Collection (ATCC, Manassas, VA, USA) and were cultured in Eagle's Minimum Essential Medium (ATCC) containing 10% FBS (Invitrogen, Carlsbad, CA, USA). Primary mouse cortical neurons (MCN) were isolated from pregnant embryonic day 18 (E18) mice as previously described.21 Briefly, mouse cerebral cortices were digested for 15 min at 37℃ using 0.25% trypsin in Hank's Balanced Salt Solution (HBSS, Corning, NY, USA) without calcium and magnesium, followed by dissociation using a fire-bored Pasteur pipette. Next, cells were maintained using DMEM medium (Invitrogen) supplemented with 5% FBS (Invitrogen) on poly-L-lysine-coated dishes. At 3 h after incubation, DMEM medium was changed to Neurobasal™ medium (Gibco, Grand Island, NY, USA) supplemented with B27 supplement (Gibco) and 0.5 mM glutamine (Gibco). All experiments were started from 6–7 days after plating. N2a and MCN were cultured in an incubation chamber with 5% CO2 at 37℃.
PPAR-γ antagonist GW9662 and PPAR-γ agonist Troglitazone (Tro) were obtained from MedChem Express (MCE, Monmouth Junction, NJ, USA). Aβ-Peptide (1–42) monomer (rat/mouse) was purchased from Abcam (Cambridge, UK). Aβ Peptide was dissolved in 1% NH4OH/Water and stored in aliquots in tightly sealed vials at −20℃. The solution was equilibrated to room temperature for at least 1 hour before use. MiR-128 mimic and its scramble control (miR-control) and miR-128 inhibitor (anti-miR-128) and its scramble control (anti-miR-control) were purchased from GenePharma Co., Ltd (Shanghai, China). These mimics or inhibitors were transfected into MCN or N2a cells by Lipofectamine 2000 reagent (Invitrogen) referring to the manufacturer's protocols.
Total RNA was extracted from plasma (1 mL) and cells using TRIzol reagent (Invitrogen) following the protocols of the manufacturer. Then, cDNA first strands were synthesized by MMLV reverse transcriptase (Invitrogen) and random primer (PPAR-γ, β-actin or U6 snRNA) or specific reverse transcription (RT) primers (miR-128). Next, PowerUp™ SYBR™ Green Master Mix (Thermo Fisher Scientific, Waltham, MA, USA) and quantified PCR (qPCR) primers were used to detect expression patterns of PPAR-γ, β-actin, miR-128, or U6 snRNA. β-actin acted as an endogenous control of PPAR-γ, and U6 snRNA served as an internal normalization standard of miR-128. RT primer sequences were as follows: miR-128, 5′-GTCGTATCCAGTG CAGGGTCCGAGGTATTCGCACTGGATACGACAAAGAG-3′. qPCR primer sequences were as follows: miR-128, 5′-TCC GATCACAGTGAACCGGT-3′ (forward) and 5′-GTG CAGGGTCCGAGGT-3′ (reverse); U6, 5′-CTCGCTTCGGCAG CACA-3′ (forward) and 5′-AACGCTTCACGAATTTGCGT-3′ (reverse); PPAR-γ, 5′-GACCTGAAACTTCAAGAGTACCAAA-3′ (forward) and 5′-TGAGGCTTATTGTAGAGCTGAGTC-3′ (reverse); β-actin 5′-AGGGGCCGGACTCGTCATACT-3′ (forward) and 5′-GGCGGCACCACCATGTACCCT-3′ (reverse).
Treated or transfected cells were collected at indicated time points, followed by the extraction of whole protein using RIPA buffer (Beyotime Biotechnology, Shanghai, China). Then, equal weight (40 µg) protein in each sample was separated by SDS-PAGE electrophoresis and then transferred to NC membranes (Millipore, Bedford, MA, USA). After blocking in 5% non-fat milk for 2 h at room temperature, the membranes were incubated with primary antibodies against PPAR-γ or β-actin (Abcam, Cambridge, UK) overnight at 4℃. Subsequently, the membranes were probed with horseradish peroxidase-conjugated appropriate secondary antibody for 1 h at room temperature. Finally, specific protein signals in the membranes were detected using Clarity™ ECL Western Blotting Substrates (Bio-Rad, Hercules, CA, USA) and quantified using Image J software (National Institutes of Health, Bethesda, MD, USA).
Cell viability was assessed by MTT (Sigma-Aldrich, St. Louis, MO, USA) assay. Transfected or treated MCN and N2a cells were plated into 96-well plates and were cultured in corresponding medium. At the indicated time after incubation, 10 µL of MTT solution (5 mg/mL) was added to each well for 4 h at 37℃. Next, generated formazan crystal was dissolved in 150 µL of DMSO (Sigma-Aldrich), followed by the detection of absorbance at 490 nm.
Cell apoptotic rate was determined using flow cytometry via an Annexin V-FITC Apoptosis Detection Kit (Sangon Biotech, Shanghai, China). Generally, cells were re-suspended in 195 µL of binding buffer (1×) at a density of 2−5×105 cells/mL. Then, 5 µL of Annexin V-FITC solution was added to each sample for 15 min at room temperature in the dark. After washing with binding buffer (1×), 10 µL of propidium iodide solution was added to cell samples [re-suspended in 195 µL of binding buffer (1×)]. Finally, cell apoptotic rate was determined by flow cytometry (BD Biosciences, San Jose, CA, USA).
Partial fragments of PPAR-γ 3′UTR containing predicted miR-128 binding sites were amplified by PCR and subcloned into pGL3-control vectors (Promega, Madison, WI, USA) to generate wild-type (WT) PPAR-γ reporter. Moreover, mutant type (MUT) PPAR-γ reporter with mutant miR-128 binding sites was produced using Quickchage Multi Site-Directed Mutagenesis kit (Stratagene, Lajolla, CA, USA). Next, constructed WT-PPAR-γ or MUT-PPAR-γ reporter was co-transfected into MCN and N2a cells together with control Renilla luciferase pRL-TK vectors (Promega) and miR-Control or miR-128. At 48 h after transfection, relative luciferase activity was determined via dualluciferase reporter assay (Promega).
Caspase 3 activity in MCN and N2a cells was measured using a Caspase 3 Activity Assay Kit (Beyotime Biotechnology) following the instructions of the manufacturer. Briefly, collected cells were lysed using lysis buffer provided in the kit, followed by high-speed centrifugation to obtain cell supernatant. Then, caspase 3 substrate Ac-DEVD-pNA (0.2 mM) was added to cell supernatant. After incubation for 2 h at 37℃, caspase 3 activity was determined at 405 nm.
MCN and N2a cells were treated as described in the figure legends. NF-κB activity was determined using NF-κB p65 Transcription Factor Assay Kit (Abcam) following the manufacturer's instructions.
Our results were obtained from at least three independent experiments and are presented as a mean±SD. Significance differences were analyzed using one-way ANOVA or Student's t-test. Differences were considered to be statistically significant when p<0.05 and to be strikingly significant when p<0.01.
Firstly, miR-128 and PPAR-γ levels in plasma from healthy volunteers (normal, n=20) and AD patients (n=20) were determined by RT-qPCR assay. MiR-128 expression was markedly increased and PPAR-γ expression was significantly decreased in the plasma from AD patients, compared to that from healthy volunteers (Fig. 1A and B), suggesting that miR-128 and PPAR-γ might play a role in AD pathogenicity. Aβ has been identified as a vital mediator in the initiation and progression of AD.2 Hence, expression patterns of miR-128 and PPAR-γ in Aβ-treated primary MCN and N2a cells were measured. As displayed in Fig. 1C and D, Aβ induced a notable increase in miR-128 expression in a dose-dependent manner in MCN and N2a cells. Moreover, PPAR-γ expression at the mRNA (Fig. 1E and F) and protein (Fig. 1G and 1H) level was remarkably reduced in a dose-dependent manner under the treatment of Aβ in primary MCN and N2a cells. Taken together, these results indicated that miR-128 and PPAR-γ might be associated with AD progression.
To further investigate the roles of miR-128 in Aβ-induced neurotoxicity, MCN and N2a cells transfected with miR-128 inhibitor (anti-miR-128) or its negative control (anti-miR-control) were treated with different doses of Aβ (0, 5, 10, 20 µM) for 24 h, followed by detection of cell viability, apoptotic rate, and caspase 3 activity. Results showed that Aβ induced a marked downregulation of cell viability and a significant upregulation of apoptotic rate and caspase-3 activity, showing the cytotoxicity of Aβ on MCN and N2a cells (Fig. 2A–F). However, the depletion of miR-128 weakened Aβ-mediated cytotoxicity in MCN and N2a cells, as reflected by increased cell viability, decreased apoptotic rate, and reduced caspase-3 activity in Aβ-treated cells following the inhibition of miR-128 by anti-miR-128 (Fig. 2A–F). Additionally, Aβ stimulation triggered a significant upregulation of NF-κB activity in MCN and N2a cells, while this effect was abated by miR-128 inhibition (Fig. 2G and H). Thus, these data showed that inhibition of miR-128 alleviates Aβ-mediated cytotoxicity by inactivating NF-κB in MCN and N2a cells.
To further explore miR-128, the Targetscan website (http://www.targetscan.org) was used to search for potential targets of miR-128. Among candidate targets, PPAR-γ was selected by virtue of its important roles in neurodegenerative disorders, including AD (Fig. 3A).22 To further validate this prediction, the effect of miR-128 on luciferase activity of WT and MUT PPAR-γ reporter was examined in primary MCN and N2a cells. Results showed that miR-128 overexpression notably reduced luciferase activity of WT PPAR-γ reporter, while it had no effect on the luciferase activity of MUT PPAR-γ reporter in MCN and N2a cells (Fig. 3B and C). These results indicated that miR-128 could directly interact with PPAR-γ 3′UTR via putative binding sites. Next, we further demonstrated that miR-128 overexpression strikingly decreased PPAR-γ expression at mRNA (Fig. 3D and E) and protein (Fig. 3F and G) levels in MCN and N2a cells. Conversely, the introduction of miR-128 inhibitor induced a significant increase in PPAR-γ expression at mRNA (Fig. 3D and E) and protein (Fig. 3F and G) levels in MCN and N2a cells. These data indicated that PPAR-γ is a target of miR-128.
Next, the PPAR-γ agonist Tro and the PPAR-γ antagonist GW 9662 were used to explore the effect of PPAR-γ on Aβ-mediated cytotoxicity in MCN and N2a cells. First, we demonstrated that the PPAR-γ agonist Tro facilitates PPAR-γ expression, while the PPAR-γ antagonist GW9662 suppresses PPAR-γ expression in MCN and N2a cells (Fig. 4A and B). Functional analyses revealed that the upregulation of PPAR-γ by Tro weakened Aβ-induced cytotoxicity in MCN and N2a cells, as reflected in increased cell viability and reduced apoptotic rate in Aβ-treated cells following the addition of Tro (Fig. 4C–F). Conversely, inactivation of PPAR-γ by GW9662 in Aβ-treated cells resulted in a dramatic decrease in cell viability and a notable increase in apoptotic rate, indicating that PPAR-γ inactivation could potentiate Aβ-mediated cytotoxicity in MCN and N2a cells. Moreover, enhanced NF-κB activity by Aβ was lessened by Tro and was improved by GW9662 (Fig. 4G and H). Thus, PPAR-γ upregulation by Tro inhibited Aβ-induced NF-κB activation, while conversely, PPAR-γ downregulation by GW9662 facilitated Aβ-triggered NF-κB activation in MCN and N2a cells (Fig. 4G and H). Collectively, these data showed that PPAR-γ attenuated Aβ-mediated cytotoxicity via inactivating NF-κB in MCN and N2a cells.
Next, we further demonstrated that depletion of PPAR-γ by GW9662 results in marked suppression of cell viability and a notable elevation of apoptotic rate in anti-miR-128-transfected and Aβ-treated MCN and N2a cells (Fig. 5A–D). In other words, PPAR-γ downregulation by GW9662 abolished the inhibitory effect of anti-miR-128 on Aβ-induced cytotoxicity in MCN and N2a cells. Additionally, the inhibition of PPAR-γ by GW9662 significantly improved NF-κB activity in anti-miR-128-transfected and Aβ-treated MCN and N2a cells (Fig. 5E and F), indicating that anti-miR-128 exerts its inhibitory effect on NF-κB activity by upregulating PPAR-γ in Aβ-treated MCN and N2a cells. Collectively, these data demonstrated that miR-128 inhibitor decreases Aβ-mediated cytotoxicity by upregulating PPAR-γ via inactivating NF-κB signaling in MCN and N2a cells.
Mounting evidence indicates that miRNAs play critical roles in the progression and pathophysiology of AD by regulating critical proteins and key biological processes, such as Aβ levels, cellular senescence, and inflammation.232425 For instance, microRNA-153 (miR-153) expression has been found to be reduced in the brains of advanced AD patients, and miR-153 has been shown to suppress APP expression in cultured human fetal brain cells.26 Others have demonstrated that microRNA-26b (miR-26b) expression is upregulated in AD and that its overexpression facilitate tau-phosphorylation and AD neuronal pathology.27
As mentioned above, abnormal miR-128 expression has been noted in the hippocampus of AD patients.1213 Moreover, downregulation of miR-128 has been found to facilitate excitability of cultured cortical neuronal networks.28 Tiribuzi, et al.29 showed that miR-128 downregulation facilitates Aβ degradation in monocytes from sporadic AD patients, indicating that miR-128 contributes to AD progression. However, Guidi, et al.30 demonstrated that miR-128 overexpression results in a marked increase in cell number and anti-apoptotic factor BCL2 levels in SH-SY5Y neuroblastoma cells. In the present study, we demonstrated that miR-128 is highly expressed in plasma from AD patients and in Aβ-treated MCN and N2a cells. Additionally, we discovered that the inhibition of miR-128 weakens Aβ-mediated cytotoxicity on MCN and N2a cells, reflected in decreased cell viability and increased apoptotic rate and caspase activity in Aβ-treated cells following depletion of miR-128 by anti-miR-128.
Previous studies have shown that NF-κB is activated in neurons surrounding early plaques and brains of AD patients.3132 Additionally, Aβ was found to induce NF-κB activation in primary neurons and neuronal cells.313233 Hence, we further tested whether miR-128 exerts its roles by regulating NF-κB activity. Our results revealed that Aβ stimulates an increase in NF-κB activity in a dose-dependent manner at concentrations of 0–20 µM in MCN and N2a cells, which is in accordance with the aforementioned studies. Furthermore, downregulation of miR-128 inhibited Aβ-induced NF-κB activation in MCN and N2a cells. Overall, this suggests that the inhibition of miR-128 weakens Aβ-mediated cytotoxicity by inhibiting NF-κB activation in MCN and N2a cells.
PPAR-γ has been shown to be a critical factor in multiple processes, such as neuroprotection, anti-inflammation, and metabolism.343536 Moreover, mounting evidence suggests that PPAR-γ could regulate lipid and glucose metabolism and inhibit inflammatory gene expression in AD. Accordingly, PPAR-γ agonists are considered attractive therapeutic targets for AD.1437 For instance, PPAR-γ agonist pioglitazone enhances cerebellar dysfunction at the pre-Aβ deposition stage in AD model mice.38 In the present study, we demonstrated that PPAR-γ expression is notably decreased in the plasma of AD patients and in Aβ-stimulated MCN and N2a cells, which is consistent with earlier findings.15 However, studies on PPAR-γ expression in AD patients are considered controversial. For instance, de la Monte and Wands39 and Kitamura, et al.40 demonstrated that PPAR-γ expression is strikingly elevated in AD brain. Meanwhile, functional analyses in the present study revealed that PPAR-γ activation by Tro weakens Aβ-induced cytotoxicity in MCN and N2a cells, similar to a preceding study.41 Conversely, PPAR-γ inhibition by GW9662 potentiated Aβ-mediated cytotoxicity in MCN and N2a cells. Prior studies also indicated that activation or inducing expression of PPAR-γ results in a marked reduction in Aβ levels in cultured neuronal and nonneuronal cells,424344 suggesting that PPAR-γ might exert protective effects on AD patients. Furthermore, upregulation of PPAR-γ by Tro hampered Aβ-induced NF-κB activation, and downregulation of PPAR-γ by GW9662 contributed to Aβ-induced NF-κB activation in MCN and N2a cells. Collectively, our data showed that PPAR-γ activation weakens Aβ-induced cytotoxicity by inactivating NF-κB signaling in MCN and N2a cells. Subsequent restoration assays further demonstrated that PPAR-γ downregulation by GW9662 abolishes the inhibitory effect of anti-miR-128 on Aβ-induced cytotoxicity by increasing NF-κB activity in MCN and N2a cells.
Taken together, our results revealed that miR-128 abates Aβ-mediated neurotoxicity by targeting PPAR-γ via inactivation of NF-κB in MCN and N2a cells. Our findings may contribute to determining the roles and molecular mechanisms of miR-128 and PPAR-γ in AD progression, providing a potential therapeutical target for AD.
Figures and Tables
Fig. 1
MiR-128 expression is upregulated and PPAR-γ expression is downregulated in plasma from AD patients and Aβ-treated MCN and N2a cells. (A and B) Expression patterns of miR-128 and PPAR-γ in plasma from healthy volunteers (Normal) (n=20) and AD patients (n=20) were detected using RT-qPCR assay. (C–H) Primary MCN cells and N2a cells were treated with different concentrations of Aβ (0, 5, 10, 20 µM) for 24 h. Then, levels of miR-128 (C and D) and PPAR-γ mRNA (E and F) were determined by RT-qPCR assay, and PPAR-γ protein expression (G and H) was measured using Western blot assay. *p<0.05. PPAR-γ, proliferator-activated receptor gamma; AD, Alzheimer's disease; Aβ, amyloid-β; MCN, mouse cortical neurons; N2a, Neuro2a; mRNA, messenger RNA.
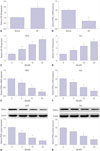
Fig. 2
Inhibition of miR-128 abates Aβ-mediated cytotoxicity by inactivating NF-κB in MCN and N2a cells. (A–H) MCN and N2a cells were transfected with antimiR-control or anti-miR-128 for 24 h and then treated with different concentrations of Aβ (0, 5, 10, 20 µM) for another 24 h, followed by detection of cell viability (A and B), apoptotic rate (C and D), caspase 3 activity (E and F), and NF-κB activity (G and H). *p<0.05, †p<0.01, ‡p<0.05, §p<0.01. Aβ, amyloid-β; MCN, mouse cortical neurons; N2a, Neuro2a.
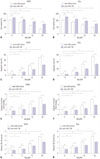
Fig. 3
PPAR-γ is a target of miR-128. (A) Putative binding sites between PPAR-γ 3′UTR and mouse miR-128, and mutant sites in MUT PPAR-γ reporter. (B and C) MCN and N2a cells were co-transfected with WT or MUT PPAR-γ reporter and miR-control or miR-128 mimic for 48 h, followed by measurement of luciferase activity via double luciferase reporter assay. (D–G) MCN and N2a cells were transfected with miR-control, miR-128 mimic, anti-miR-control, or anti-miR-128 for 48 h. Then, PPAR-γ expressions at mRNA (D and E) and protein (F and G) levels were determined by RT-qPCR and Western blot assays, respectively. *p<0.05. PPAR-γ, proliferator-activated receptor gamma; MCN, mouse cortical neurons; N2a, Neuro2a; mRNA, messenger RNA; WT, wild type; MUT, mutant type.
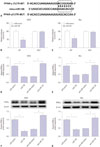
Fig. 4
PPAR-γ attenuates Aβ-mediated cytotoxicity by inactivating NF-κB in MCN and N2a cells. (A and B) MCN and N2a cells were treated with Control (DMSO), Tro (20 µM), or GW9662 (10 µM) for 24 h, followed by detection of PPAR-γ protein level via Western blot assay. (C–H) MCN and N2a cells were treated with Aβ (10 µM) for 24 h and stimulated with Control (DMSO), Tro (20 µM), or GW9662 (10 µM) for another 24 h. Next, at the indicated time point, cell viability (C and D), apoptotic rate (E and F), and NF-κB activity (G and H) were determined. *p<0.05. PPAR-γ, proliferator-activated receptor gamma; Aβ, amyloid-β; MCN, mouse cortical neurons; N2a, Neuro2a; Tro, troglitazone.
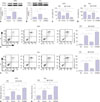
Fig. 5
miR-128 inhibitor decreases Aβ-mediated cytotoxicity by upregulating PPAR-γ via inactivation of NF-κB signaling in MCN and N2a cells. (A–F) MCN and N2a cells were treated with Aβ (10 µM) for 24 h, followed by stimulation of control (DMSO) or Tro (20 µM) for another 24 h. Aβ-treated cells were transfected with anti-miR-control or anti-miR-128 for another 24 h, together with or without the treatment of control (DMSO) or GW9662 (10 µM) for an additional 24 h. Following this, cell viability (A and B), apoptotic rate (C and D), and NF-κB activity (E and F) were determined in treated cells. *p<0.05. Aβ; amyloid-β PPAR-γ, proliferator-activated receptor gamma; MCN, mouse cortical neurons; N2a, Neuro2a; Tro, troglitazone.
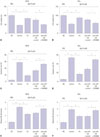
References
1. Alzheimer's Association. 2016 Alzheimer's disease facts and figures. Alzheimers Dement. 2016; 12:459–509.
2. Reitz C, Mayeux R. Alzheimer disease: epidemiology, diagnostic criteria, risk factors and biomarkers. Biochem Pharmacol. 2014; 88:640–651.


3. Kumar A, Singh A, Ekavali . A review on Alzheimer's disease pathophysiology and its management: an update. Pharmacol Rep. 2015; 67:195–203.


4. Kim DH, Yeo SH, Park JM, Choi JY, Lee TH, Park SY, et al. Genetic markers for diagnosis and pathogenesis of Alzheimer's disease. Gene. 2014; 545:185–193.


5. Selkoe DJ, Hardy J. The amyloid hypothesis of Alzheimer's disease at 25 years. EMBO Mol Med. 2016; 8:595–608.


6. Kang S, Lee YH, Lee JE. Metabolism-Centric Overview of the Pathogenesis of Alzheimer's Disease. Yonsei Med J. 2017; 58:479–488.


7. Mémet S. NF-kappaB functions in the nervous system: from development to disease. Biochem Pharmacol. 2006; 72:1180–1195.
8. Shi ZM, Han YW, Han XH, Zhang K, Chang YN, Hu ZM, et al. Upstream regulators and downstream effectors of NF-κB in Alzheimer's disease. J Neurol Sci. 2016; 366:127–134.


9. Cai Y, Yu X, Hu S, Yu J. A brief review on the mechanisms of miRNA regulation. Genomics Proteomics Bioinformatics. 2009; 7:147–154.


10. Karnati HK, Panigrahi MK, Gutti RK, Greig NH, Tamargo IA. miRNAs: key players in neurodegenerative disorders and epilepsy. J Alzheimers Dis. 2015; 48:563–580.


11. Adlakha YK, Saini N. Brain microRNAs and insights into biological functions and therapeutic potential of brain enriched miRNA-128. Mol Cancer. 2014; 13:33.


12. Lukiw WJ. Micro-RNA speciation in fetal, adult and Alzheimer's disease hippocampus. Neuroreport. 2007; 18:297–300.


13. Müller M, Kuiperij HB, Claassen JA, Küsters B, Verbeek MM. MicroRNAs in Alzheimer's disease: differential expression in hippocampus and cell-free cerebrospinal fluid. Neurobiol Aging. 2014; 35:152–158.


14. Jiang Q, Heneka M, Landreth GE. The role of peroxisome proliferator-activated receptor-gamma (PPARgamma) in Alzheimer's disease: therapeutic implications. CNS Drugs. 2008; 22:1–14.


15. Sastre M, Dewachter I, Rossner S, Bogdanovic N, Rosen E, Borghgraef P, et al. Nonsteroidal anti-inflammatory drugs repress beta-secretase gene promoter activity by the activation of PPARgamma. Proc Natl Acad Sci U S A. 2006; 103:443–448.


16. Combs CK, Johnson DE, Karlo JC, Cannady SB, Landreth GE. Inflammatory mechanisms in Alzheimer's disease: inhibition of beta-amyloid-stimulated proinflammatory responses and neurotoxicity by PPARgamma agonists. J Neurosci. 2000; 20:558–567.


17. Fakhfouri G, Ahmadiani A, Rahimian R, Grolla AA, Moradi F, Haeri A. WIN55212-2 attenuates amyloid-beta-induced neuroinflammation in rats through activation of cannabinoid receptors and PPAR-γ pathway. Neuropharmacology. 2012; 63:653–666.


18. Dubois B, Feldman HH, Jacova C, Hampel H, Molinuevo JL, Blennow K, et al. Advancing research diagnostic criteria for Alzheimer's disease: the IWG-2 criteria. Lancet Neurol. 2014; 13:614–629.


19. Schmidt K. Clinical dementia rating scale. In : Michalos AC, editor. Encyclopedia of quality of life and well-being research. Dordrecht: Springer;2014. p. 957–960.
20. Mitchell AJ. The Mini-Mental State Examination (MMSE): update on its diagnostic accuracy and clinical utility for cognitive disorders. In : Larner AJ, editor. Cognitive screening instruments. Cham: Springer;2017. p. 37–48.
21. Krichevsky AM, Kosik KS. Neuronal RNA granules: a link between RNA localization and stimulation-dependent translation. Neuron. 2001; 32:683–696.
22. Chen YC, Wu JS, Tsai HD, Huang CY, Chen JJ, Sun GY, et al. Peroxisome proliferator-activated receptor gamma (PPAR-γ) and neurodegenerative disorders. Mol Neurobiol. 2012; 46:114–124.


23. Reddy PH, Tonk S, Kumar S, Vijayan M, Kandimalla R, Kuruva CS, et al. A critical evaluation of neuroprotective and neurodegenerative MicroRNAs in Alzheimer’s disease. Biochem Biophys Res Commun. 2017; 483:1156–1165.


24. Femminella GD, Ferrara N, Rengo G. The emerging role of microRNAs in Alzheimer's disease. Front Physiol. 2015; 6:40.


25. Millan MJ. Linking deregulation of non-coding RNA to the core pathophysiology of Alzheimer's disease: an integrative review. Prog Neurobiol. 2017; 156:1–68.


26. Long JM, Ray B, Lahiri DK. MicroRNA-153 physiologically inhibits expression of amyloid-β precursor protein in cultured human fetal brain cells and is dysregulated in a subset of Alzheimer disease patients. J Biol Chem. 2012; 287:31298–31310.


27. Absalon S, Kochanek DM, Raghavan V, Krichevsky AM. MiR-26b, upregulated in Alzheimer’s disease, activates cell cycle entry, tauphosphorylation, and apoptosis in postmitotic neurons. J Neurosci. 2013; 33:14645–14659.


28. McSweeney KM, Gussow AB, Bradrick SS, Dugger SA, Gelfman S, Wang Q, et al. Inhibition of microRNA 128 promotes excitability of cultured cortical neuronal networks. Genome Res. 2016; 26:1411–1416.


29. Tiribuzi R, Crispoltoni L, Porcellati S, Di Lullo M, Florenzano F, Pirro M, et al. miR128 up-regulation correlates with impaired amyloid β(1-42) degradation in monocytes from patients with sporadic Alzheimer's disease. Neurobiol Aging. 2014; 35:345–356.


30. Guidi M, Muiños-Gimeno M, Kagerbauer B, Martí E, Estivill X, Espinosa-Parrilla Y. Overexpression of miR-128 specifically inhibits the truncated isoform of NTRK3 and upregulates BCL2 in SHSY5Y neuroblastoma cells. BMC Mol Biol. 2010; 11:95.


31. Kaltschmidt B, Uherek M, Volk B, Baeuerle PA, Kaltschmidt C. Transcription factor NF-kappaB is activated in primary neurons by amyloid beta peptides and in neurons surrounding early plaques from patients with Alzheimer disease. Proc Natl Acad Sci U S A. 1997; 94:2642–2647.


32. Valerio A, Boroni F, Benarese M, Sarnico I, Ghisi V, Bresciani LG, et al. NF-kappaB pathway: a target for preventing beta-amyloid (Abeta)-induced neuronal damage and Abeta42 production. Eur J Neurosci. 2006; 23:1711–1720.


33. Lin W, Ding M, Xue J, Leng W. The role of TLR2/JNK/NF-κB pathway in amyloid β peptide-induced inflammatory response in mouse NG108-15 neural cells. Int Immunopharmacol. 2013; 17:880–884.


34. Villapol S. Roles of peroxisome proliferator-activated receptor gamma on brain and peripheral inflammation. Cell Mol Neurobiol. 2018; 38:121–132.


35. Corona JC, Duchen MR. PPARγ as a therapeutic target to rescue mitochondrial function in neurological disease. Free Radic Biol Med. 2016; 100:153–163.


36. Lezana JP, Dagan SY, Robinson A, Goldstein RS, Fainzilber M, Bronfman FC, et al. Axonal PPARγ promotes neuronal regeneration after injury. Dev Neurobiol. 2016; 76:688–701.


37. Landreth G, Jiang Q, Mandrekar S, Heneka M. PPARgamma agonists as therapeutics for the treatment of Alzheimer's disease. Neurotherapeutics. 2008; 5:481–489.


38. Toba J, Nikkuni M, Ishizeki M, Yoshii A, Watamura N, Inoue T, et al. PPARγ agonist pioglitazone improves cerebellar dysfunction at pre-Aβ deposition stage in APPswe/PS1dE9 Alzheimer's disease model mice. Biochem Biophys Res Commun. 2016; 473:1039–1044.


39. de la Monte SM, Wands JR. Molecular indices of oxidative stress and mitochondrial dysfunction occur early and often progress with severity of Alzheimer's disease. J Alzheimers Dis. 2006; 9:167–181.


40. Kitamura Y, Shimohama S, Koike H, Kakimura Ji, Matsuoka Y, Nomura Y, et al. Increased expression of cyclooxygenases and peroxisome proliferator-activated receptor-gamma in Alzheimer's disease brains. Biochem Biophys Res Commun. 1999; 254:582–586.


41. Inestrosa NC, Godoy JA, Quintanilla RA, Koenig CS, Bronfman M. Peroxisome proliferator-activated receptor gamma is expressed in hippocampal neurons and its activation prevents beta-amyloid neurodegeneration: role of Wnt signaling. Exp Cell Res. 2005; 304:91–104.


42. Sastre M, Dewachter I, Landreth GE, Willson TM, Klockgether T, van Leuven F, et al. Nonsteroidal anti-inflammatory drugs and peroxisome proliferator-activated receptor-gamma agonists modulate immunostimulated processing of amyloid precursor protein through regulation of beta-secretase. J Neurosci. 2003; 23:9796–9804.


43. Camacho IE, Serneels L, Spittaels K, Merchiers P, Dominguez D, De Strooper B. Peroxisome-proliferator-activated receptor gamma induces a clearance mechanism for the amyloid-beta peptide. J Neurosci. 2004; 24:10908–10917.


44. d'Abramo C, Massone S, Zingg JM, Pizzuti A, Marambaud P, Dalla Piccola B, et al. Role of peroxisome proliferator-activated receptor gamma in amyloid precursor protein processing and amyloid beta-mediated cell death. Biochem J. 2005; 391(Pt 3):693–698.