Abstract
In this study, we demonstrated the inhibitory effect of the Class Ic antiarrhythmic agent propafenone on voltage-dependent K+ (Kv) channels using freshly isolated coronary artery smooth muscle cells from rabbits. The Kv current amplitude was progressively inhibited by propafenone in a dose-dependent manner, with an apparent IC50 value of 5.04±1.05 µM and a Hill coefficient of 0.78±0.06. The application of propafenone had no significant effect on the steady-state activation and inactivation curves, indicating that propafenone did not affect the voltage-sensitivity of Kv channels. The application of train pulses at frequencies of 1 or 2 Hz progressively increased the propafenone-induced inhibition of the Kv current. Furthermore, the inactivation recovery time constant was increased after the application of propafenone, suggesting that the inhibitory action of propafenone on Kv current is partially use-dependent. Pretreatment with Kv1.5, Kv2.1 or Kv7 inhibitor did not change the inhibitory effect of propafenone on the Kv current. Together, these results suggest that propafenone inhibits the vascular Kv channels in a dose- and use-dependent manner, regardless of Na+ channel inhibition.
Propafenone, a Class Ic antiarrhythmic agent, is used widely for the treatment of supraventricular and ventricular tachycardia as a potent inhibitor of sodium channels and it also prevents recurrence in patients with atrial fibrillation without structural cardiac disease [1]. Propafenone binds to the activated receptor site of the Na+ channel and dissociates slowly from its combined site, which decreases the velocity of action potential transmission, thereby serving to suppress tachycardia induced by abnormal rapid conduction [2]. Although propafenone is clinically useful for the treatment of arrhythmia, it has been found to affect other channels in addition to having Na+ channel-blocking properties. For example, propafenone inhibits Ca2+ channels recorded from isolated guinea-pig ventricular myocytes [3]. Furthermore, it has been reported to inhibit K+ channels in rabbit atrial myocytes [4], guinea-pig ventricular myocytes [5], and vascular smooth muscle from rat portal veins [6]. However, the detailed inhibition mechanisms of propafenone on ion channels, specifically voltage-dependent K+ (Kv) channels, have not been studied in arterial smooth muscle cells.
Several types of K+ channel, including ATP-sensitive K+ (KATP), large-conductance Ca2+-activated K+ (BKCa), inward rectifier K+ (Kir), and Kv channels, have been identified in arterial smooth muscle cells [7]. Although each K+ channel contributes to the maintenance of resting membrane potential and resting tone, Kv channels are most highly expressed in arterial smooth muscle, and the inhibition of Kv channels induces membrane depolarization in some arteries [891011]. Furthermore, loss of Kv channel function has been found to be associated with various cardiovascular and metabolic diseases [121314]. For this reason, studies of the changes in arterial Kv channel function and/or expression are essential for the understanding of vascular function and vascular-related diseases. In fact, coronary artery plays a role in supplying blood to the heart, and the coronary arterial dysfunction through the inhibition of the Kv channel leads to a reduction in oxygen and nutrient supply to the cardiac muscle, resulting in severe cardiovascular diseases. Therefore, considering the efficacy of propafenone for the treatment of arrhythmia and the pathophysiological significance of coronary arterial Kv channels, studies of the effect of propafenone on coronary arterial Kv channels are important for the accurate administration of propafenone in patients with both arrhythmia and cardiovascular disease.
In this study, we investigated the effect of propafenone on vascular KV channels using freshly isolated rabbit coronary arterial smooth muscle cells. Our study clearly showed that propafenone inhibited coronary arterial Kv channels in a dose- and use-dependent manner, regardless of Na+ channel inhibition.
All animal experimental procedures were performed in accordance with guidelines established by the Committee for Animal Experiments at Kangwon National University. Male New Zealand White rabbits (1.7–2.2 kg) were anesthetized by administration of a pentobarbitone (75 mg/kg) and heparin sodium (120 U/kg) mixture through the ear vein. The thoracic cavity was dissected and the heart was rapidly removed and then transferred to normal Tyrode's solution. The second branches of coronary arteries were carefully isolated from the heart, and cleaned of surrounding tissues under a stereoscope. The endothelium was eliminated by flowing an air bubble into the lumen of each coronary arteries. Single coronary artery smooth muscle cells were isolated using a two-step enzymatic procedure. The first enzymatic treatment was performed by submerging coronary arteries in 1 mL Ca2+-free normal Tyrode's solution containing papain (1.3 mg/mL), bovine serum albumin (BSA, 1.6 mg/mL), and dithiothreitol (DTT, 2.0 mg/mL) for 25 min at 36.8℃. After washing in Ca2+-free normal Tyrode's solution, the arteries were transferred to Ca2+-free normal Tyrode's solution containing collagenase (2.6 mg/mL), BSA (1.6 mg/mL), and DTT (2.0 mg/mL) for 18 min at 36.8℃. Enzyme-treated arteries were carefully agitated using a fire-polished Pasture pipette to obtain single smooth muscle cells. The isolated single cells were stored at 4℃ in Kraft-Brühe (KB) solution and used within 10 h.
The normal Tyrode's solution contained the following (in mM): 133 NaCl, 5.2 KCl, 0.36 NaH2PO4, 15.6 glucose, 5.5 HEPES, 0.7 MgCl2, 1.8 CaCl2, adjusted to pH 7.35 with NaOH. The KB solution contained the following (in mM): 10 HEPES, 24 L-glutamate, 17 taurine, 68 KH2PO4, 16 glucose, 0.6 EGTA, 2.5 MgCl2, 52 KCl, 50 KOH, adjusted to pH 7.30 with KOH. The pipette-filling solution contained the following (in mM): 113 K-aspartate, 3.6 Mg-ATP, 8 EGTA, 10 HEPES, 2.5 MgCl2, 4.6 NaCl, 27 KCl, adjusted to pH 7.25 with KOH. Propafenone was purchased from Sigma Chemical Co. (St. Louis, MO, USA) and dissolved in dimethyl sulfoxide (DMSO). Diphenyl phosphine oxide-1 (DPO-1), guangxitoxin, and linopirdine were purchased from Tocris Cookson (Ellisville, MO, USA) and dissolved in dimethyl sulfoxide (DMSO).
Whole-cell currents were measured from single coronary artery smooth muscle cells using a patch-clamp amplifier (Axopatch 200B; Axon Instruments, Union City, CA, USA). PatchPro software was used for pulse generationand data acquisition. Patch pipettes were prepared from borosilicate tubes using a PP-830 vertical puller (Narishige Scientific Instrument Laboratory, Tokyo, Japan). The patch pipettes exhibited a resistance of 3–4 MΩ when filled with the pipette-filling solution.
Data analysis was conducted using Origin 7.5 software (Microcal Software, Inc., Northampton, MA, USA). A first-order blocking model was applied to describe the channel-drug interaction kinetics [1516]. The IC50 value and Hill coefficient (n) were calculated using concentration-dependent data, and the values were obtained using the Hill equation:
where f indicates the fractional suppression of current (f=1−Idrug/Icontrol) at the test potential, and [D] indicates the propafenone concentration.
Tail currents, which were induced by a returning potential of −40 mV after short (20–50 ms ms) depolarizing steps from −80 to +60 mV in 10 mV increments, were used to obtain the steady-state activation curves. The recorded tail currents for each potential were normalized to the maximal tail current. Activation curves were yielded using the Boltzmann equation:
where V is the test potential, V1/2 is the potential for the half-activation point, and k is the slope value.
A two-step voltage protocol was applied to obtain the steady-state inactivation curves. Currents were recorded with a 600-ms test potential of +40 mV after 7-s pre-conditioning steps from −80 mV to +30 mV (10 mV increments) in the absence and presence of propafenone. Inactivation curves were yielded using another Boltzmann equation:
where V is the test potential, V1/2 is the potential for the half-inactivation point, and k is the slope value.
All data are presented as the mean±standard error of the mean. Student's t-test was used to determine statistical significance, and p values <0.05 were considered statistically significant.
Kv currents, which were expressed in coronary arterial smooth muscle cells, were recorded with the whole-cell patch clamp technique. The Kv currents were elicited by applying 600-ms step depolarizing pulses from −80 to +60 mV at a holding potential of −80 mV. To record only the Kv current, other K+ channels were suppressed by including EGTA (to block BKCa channels) and ATP (to block KATP channels) in the intracellular solution. Furthermore, the involvement of Kir channels was excluded by using the second branches of coronary arteries, as Kir channels have not been found in these branches. Fig. 1A shows the control Kv currents, which rapidly rose to a peak, followed by slow and partial inactivation. Application of 10 µM propafenone induced inhibition of Kv currents (Fig. 1B). This inhibition was reached within 2 min and partially (~60%) washed out. Fig. 1C shows the steady-state current-voltage (I–V) relationships in the absence and presence of 10 µM propafenone. Application of 10 µM propafenone decreased the Kv currents by 51%, as measured at +60 mV.
To determine whether the inhibition of Kv currents by propafenone was concentration-dependent, several concentrations (0, 0.1, 1, 3, 10, 30, and 100 µM) of propafenone were applied to the external solution. The Kv currents were recorded by applying a repetitive one-step depolarizing pulse to +60 mV from a holding potential of −80 mV. The inhibition of Kv current increased progressively with the concentration of propafenone (Fig. 2A). The concentration dependence of Kv channel inhibition by propafenone was analyzed using the Hill equation, as described in the Materials and Methods section. From the fit of the Hill equation, we obtained an IC50 value of 5.04±1.05 µM and a slope value (Hill coefficient) of 0.78±0.06 (Fig. 2B).
To evaluate whether the inhibitory effect of propafenone affects the gating properties of the Kv channels, we tested the effect of propafenone on the steady-state activation and inactivation kinetics of Kv currents. As described in the Materials and Methods section, the steady-state activation curve was obtained from the tail currents and the data were fitted with the Boltzmann equation. Application of 10 µM propafenone did not affect the steady state activation curve (Fig. 3A). The potentials for the half-activation point (V1/2) and slope value (k) were −10.03±1.36 mV and 16.44±1.01, respectively, under control conditions, and −8.97±0.95 mV and 15.72±0.98, respectively, in the presence of 10 µM propafenone.
We also tested the influence of propafenone on the steady-state inactivation curve. The steady-state curve was acquired using a two-step voltage protocol as described in the Materials and Methods section. Similar to the results for the activation curve, propafenone did not change the steady-state inactivation curve (Fig. 3B). The potentials for the half-inactivation point (V1/2) and slope value (k) were −27.67±0.47 mV and 2.52±0.42, respectively, under control conditions, and −28.82±0.63 mV and 3.01±1.46, respectively, in the presence of 10 µM propafenone. These data suggest that propafenone does not affect the gating properties of Kv channels.
To determine whether the inhibitory effect of propafenone on Kv channels was use-dependent, we repetitively elicited Kv currents with +60 mV pulses 20 times at frequencies of 1 and 2 Hz. The amplitudes of the Kv currents measured at the 20th pulse were slightly (10% and 17%) lower at 1 and 2 Hz, respectively, than those induced by the first pulse (Figs. 4A and B). In the presence of 10 µM propafenone, the inhibition of Kv currents was increased to 16% and 25% at 1 and 2 Hz, respectively. The overlapping raw traces of Kv currents elicited with trains of voltage steps together with the each Kv current trace elicited by the 1st, 2nd, and 3rd voltage steps are shown in the upper panels of Figs. 4A and B. The first depolarizing voltage step evoked most of the steady inhibition of the Kv current, indicating that the propafenone-induced inhibition of Kv current is largely through state-independent way. However, some small but sizable portion of the use-dependent inhibition was also evident. From these results, we concluded that propafenone inhibited the Kv current primarily through state-independent manner together with some portion of use-dependency.
To further evaluate the use-dependent inhibition of Kv currents by propafenone, we examined recovery times from the inactivation of Kv currents in the absence and presence of propafenone. During the 150 ms-depolarizing voltage-steps, small but sizable portion of Kv channels are inactivated, and the gradual decline of the Kv currents by the trains of voltage steps shown in Fig. 4 reflects the accumulation of this small portion of inactivation. Therefore, the experiment in Fig. 5 was designed with the same duration of voltage steps to examine recovery time courses of the Kv currents after the 150 ms-voltage steps in the absence and presence of propafenone. The recovery kinetics of the Kv currents under the control conditions and in the presence of 10 µM propafenone were measured using a double-pulse protocol as described in Fig. 5 inset. The interval time was increased from 30 to 7,000 ms. As shown in Fig. 5, the recovery time course was slowed in the presence of propafenone. Data were adapted to a single exponential function, yielding recovery time constants of 1,391.17±267.91 ms under control conditions and 1,741.45±181.74 ms in the presence of 10 µM propafenone. The recovery time constants were statistically significant. This state-dependent effect of propafenone (i.e., retardation of recovery from inactivation after 150 ms-depolarizing pulse) may contribute to the use-dependency shown in Fig. 4.
To determine the major Kv subtypes involved in propafenone-induced inhibition of Kv channels, we pre-treated with Kv1.5 inhibitor (DPO-1, 1 µM), Kv2.1 inhibitor (guangxitoxin, 30 nM), or Kv7 inhibitor (linopirdine, 10 µM) before applying propafenone. The application of DPO-1 reduced the Kv current by ~40% (Fig. 6A). However, the additional application of propafenone did not change the degree of inhibition (Fig. 6B, 56.2% inhibition). Similar to the results for DPO-1, the application of guangxitoxin and linopirdine immediately decreased the Kv current by 22% and 14%, respectively (Figs. 6C and E). The additional application of propafenone induced further inhibition; however, the degree of further inhibition did not differ from that of propafenone alone (Fig. 6D, 49.9% inhibition and Fig. 6F, 43.1% inhibition vs. propafenone alone, 49.4% inhibition). These results suggest that the major targets of inhibitory action by propafenone are not of the Kv1.5, Kv2.1, or Kv7 subtype.
This study was the first to investigate the effects of propafenone on Kv channels in rabbit coronary arterial smooth muscle cells. Our findings indicate that propafenone, a class Ic antiarrhythmic agent, inhibits vascular Kv channels in a dose- and use-dependent manner regardless of Na+ channel inhibition. In addition, application of propafenone did not change the activation and inactivation curves suggesting that propafenone does not affect the gating properties of Kv channels.
Our results demonstrated that propafenone inhibited vascular Kv channels regardless of its own function as an Na+ channel blocker. First, the inhibitory effect of propafenone on Kv channels reached a steady-state within 2 min. The rapid action of propafenone on the vascular Kv current could be explained by direct interaction between propafenone and the channel, not by a complicated signaling mechanism. Second, the primary target of class Ic antiarrhythmic drugs, the Na+ channel, is not present or prominent in vascular smooth muscle cells [17]. Given these facts, we conclude that the inhibitory effect of propafenone on vascular Kv channels is not mediated by Na+ channel inhibition but is more likely due to direct interaction between propafenone and Kv channels. Furthermore, the results in Fig. 4 suggest that propafenone inhibited the Kv channels largely through state-independent way together with some use-dependency. The small but sizable use-dependency seems to be arisen from the retardation of the recovery from inactivation of Kv channels in the presence of propafenone (Fig. 5). It could be inferred that this use- or state-dependent inhibition of Kv channels by propafenone could be get more important under clinical setting because propafenone can exert a stronger Kv inhibition, if any, in the areas of pre-existing coronary arterial depolarization or contraction (such as at area of coronary spasm in variant angina).
Propafenone, a class Ic antiarrhythmic agent, is well known as a potent sodium channel blocking drug [2]. It is effective in the medical treatment of supraventricular tachyarrhythmia [1]. However, administration of propafenone has been reported to be associated with cardiac toxicity, such as abnormal QT prolongation and torsade de pointes arrhythmia [1819]. Propafenone has also been speculated to cause prolongation of the action potential duration, probably due to the inhibition of cardiac K+ channels. In fact, several studies have suggested that propafenone inhibits the K+ channels expressed in atrial and ventricular myocytes in rabbits, guinea pigs, rats, and humans [452021]. Propafenone has also been reported to inhibit several types of potassium channel, including Kv, KA (the voltage-dependent transient K+channel), BKCa, and KATP channels in rat portal vein smooth muscle cells [6]. Although several studies have been performed to demonstrate the inhibitory effects of propafenone on broad types of K+ channel, the effects of propafenone on arterial K+ channels, especially the Kv channel in native coronary arterial smooth muscle cells, have not been investigated. In this study, we first identified the effect of propafenone on Kv channels in coronary arterial smooth muscle cells. Our results provide increased knowledge about the side effects of propafenone on ion channels and may contribute to the formation of safety guidelines for propafenone use in patients with arrhythmia.
Numerous studies have suggested that vascular Kv channels play an important role in maintaining basal vascular tone by regulating the resting membrane potential [7]. Furthermore, the alteration of Kv channel function and/or expression has been observed in various pathological conditions, including hypertension, hypoxia, and diabetes [12]. For this reason, the adverse effects of some drugs on vascular Kv channels should be investigated to avoid misinterpretation of clinical/experimental data regarding vascular function. To date, various drugs and chemicals have been reported to inhibit vascular Kv channels independently of their intrinsic functions [2223]. Our group recently reported the direct effect of amiodarone, a class III antiarrhythmic drug, on vascular Kv channels using coronary arterial smooth muscle cells [24]. Similar to the results of this study, amiodarone inhibited the Kv current in a dose- and use-dependent manner. However, unlike the results of this study, amiodarone shifted the inactivation curves toward a more negative potential. To date, the inhibitory effects of class Ic antiarrhythmic drugs on vascular Kv channels have not been reported. Therefore, more intensive studies should be conducted to examine the effects of the antiarrhythmic drugs on ion channels.
To date, a number of Kv subtypes, including Kv1.1, Kv1.2, Kv1.4, Kv1.5, Kv2.1, Kv7 and Kv9.3, have been identified in vascular smooth muscle cells [2526]. Among these, Kv1.5 and Kv2.1 subtypes are known to be expressed in rabbit vascular smooth muscle cells [2728]. In addition, more recently, Kv7 subtypes have been shown to modulate the resting membrane potential and resting tone [2930]. For this reason, the involvement of these subtypes in the propafenone-induced inhibition of Kv channels was investigated using specific inhibitors. However, our results provide no evidence for the involvement of these subtypes. Although we could not clearly address the exact Kv subunits involved in the propafenone effect, propafenone may inhibit multiple Kv subtypes other than Kv1.5, Kv2.1, and Kv7. To examine this issue, additional experiments should be performed using expression systems for specific Kv subtypes in the near future.
Clinically, propafenone is prescribed in 225 to 425 mg doses twice daily [31]. After taking 300 mg propafenone, the plasma concentration could reach a peak value of 0.39 µM within 2.5 h. Furthermore, therapeutic plasma concentrations of propafenone could increase to a maximum of 5.27 µM [32]. In this study, the IC50 value for the inhibition of Kv channels by propafenone was 5.04±1.05 µM, which is similar to the therapeutic plasma peak concentration of propafenone. Therefore, overmedication or abuse of propafenone can increase plasma levels up to 5 µM, which can affect the Kv channels in coronary arterial smooth muscle cells. Furthermore, application of nanomolar propafenone inhibited the Kv current (Fig. 2). Considering that vascular smooth muscle has a high input resistance, changes of only a few currents could produce changes in the resting membrane potential, and thereby vascular tone and blood flow [33]. Therefore, overmedication or abuse should be strictly prohibited when prescribing propafenone to patients with arterial diseases.
In summary, we investigated the inhibitory effect of propafenone on vascular Kv channels using rabbit coronary artery smooth muscle cells. Our results suggest that propafenone inhibits the arterial Kv channels in a dose- and use-dependent manner, regardless of intended function. Therefore, our findings should be considered when prescribing propafenone to patients with arrhythmia.
Figures and Tables
Fig. 1
The inhibitory effect of propafenone on voltage-dependent K+ (Kv) channel currents in freshly isolated rabbit coronary arterial smooth muscle cells.
The Kv currents were recorded using 600-ms step depolarizing pulses from −80 to +60 mV at a holding potential of −80 mV in the absence (A) and presence (B) of 10 µM propafenone. (C) The current-voltage (I–V) relationships for the steady-state Kv current in the absence (○) and presence (●) of 10 µM propafenone. n=9. n means cell number isolated from different rabbits. *p<0.05.
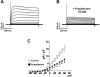
Fig. 2
Concentration-dependence of propafenone-induced Kv channel blocking.
(A) Representative Kv current traces were recorded by 600-ms one-step depolarizing pulses of +60 mV from a holding potential of −80 mV in the presence of 0, 0.1, 1, 3, 10, 30, and 100 µM propafenone. (B) Concentration-dependent curve for the inhibitory action of propafenone on Kv channel currents. The normalized currents were fitted with the Hill equation. All n=13. n means cell number isolated from different rabbits.
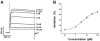
Fig. 3
Voltage-dependence of the steady-state activation and inactivation of Kv currents.
(A) Activation curves in the absence (○) and presence (●) of 10 µM propafenone. Tail currents for the activation curves were obtained by applying short (20–50 ms ms) depolarizing pulses from −80 to +60 mV in steps of 10 mV at a holding potential of −80 mV, and then returned to −40 mV. The recorded currents were normalized to the peak tail current. n=10. n means cell number isolated from different rabbits. (B) Inactivation curves in the absence (○) and presence (●) of 10 µM propafenone. The currents were obtained by a test step to +40 mV after 7-s preconditioning pulses from −80 to +30 mV. The recorded currents were normalized to the peak current elicited by pre-pulses. n=6. n means cell number isolated from different rabbits.
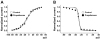
Fig. 4
Use-dependent blockage of Kv currents by propafenone.
Twenty repetitive depolarizing pulses of +60 mV from a holding potential of −80 mV were applied in the absence (○) and presence (●) of 10 µM propafenone, at frequencies of 1 Hz (A) and 2 Hz (B). The elicited peak currents were normalized by the peak current at first pulse and plotted against the pulse number. n=7. n means cell number isolated from different rabbits. *p<0.05.
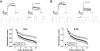
Fig. 5
Effects of propafenone on recovery time from Kv channel inactivation.
The recovery time constant was measured by applying double-step pulses as shown in the inset. The first 150 ms depolarizing pulse of +60 mV from a holding potential of −80 mV was followed by the second (same) pulse with extension of the time interval (from 30 to 7,000 ms). The solid line indicates the recovery kinetics of the Kv current recorded in the absence (○) and the presence (●) of 10 µM propafenone. n=7. n means cell number isolated from different rabbits. *p<0.05.
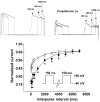
Fig. 6
The involvement of Kv1.5, Kv2.1, and Kv7 subtypes in the propafenone-induced inhibition of Kv channels.
The Kv current traces were recorded by one-step depolarizing pulses of +60 mV from a holding potential of −80 mV. (A) Superimposed Kv currents under control, in the presence of 1 µM DPO-1, and in the presence of 10 µM propafenone+1 µM DPO-1. (B) Summary of panel (A). n=5. n means cell number isolated from different rabbits. *p<0.05. (C) Representative Kv currents under control, in the presence of 30 nM guangxitoxin, and in the presence of 10 µM propafenone+30 nM guangxitoxin. (D) Summary of panel (C). n=5. n means cell number isolated from different rabbits. *p<0.05. (E) Kv current traces under control, in the presence of 10 µM linopirdine, and in the presence of 10 µM propafenone+10 µM linopirdine. (F) Summary of panel (E). n=5. n means cell number isolated from different rabbits. *p<0.05.
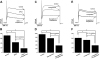
ACKNOWLEDGEMENTS
This work was supported by the National Research Foundation of Korea (NRF) grant funded by the Korea government (Ministry of Education: 2016-R1D1A3B03930169).
Notes
References
1. Kishore AG, Camm AJ. Guidelines for the use of propafenone in treating supraventricular arrhythmias. Drugs. 1995; 50:250–262.


2. Benz I, Kohlhardt M. Responsiveness of cardiac Na+ channels to antiarrhythmic drugs: the role of inactivation. J Membr Biol. 1991; 122:267–278.
3. Delgado C, Tamargo J, Henzel D, Lorente P. Effects of propafenone on calcium current in guinea-pig ventricular myocytes. Br J Pharmacol. 1993; 108:721–727.


4. Duan D, Fermini B, Nattel S. Potassium channel blocking properties of propafenone in rabbit atrial myocytes. J Pharmacol Exp Ther. 1993; 264:1113–1123.
5. Delpón E, Valenzuela C, Pérez O, Casis O, Tamargo J. Propafenone preferentially blocks the rapidly activating component of delayed rectifier K+ current in guinea pig ventricular myocytes. Voltage-independent and time-dependent block of the slowly activating component. Circ Res. 1995; 76:223–235.
6. Cogolludo AL, Pérez-Vizcaíno F, López-López G, Ibarra M, Zaragozá-Arnáez F, Tamargo J. Propafenone modulates potassium channel activities of vascular smooth muscle from rat portal veins. J Pharmacol Exp Ther. 2001; 299:801–810.
7. Nelson MT, Quayle JM. Physiological roles and properties of potassium channels in arterial smooth muscle. Am J Physiol. 1995; 268:C799–C822.


8. Leblanc N, Wan X, Leung PM. Physiological role of Ca2+-activated and voltage-dependent K+ currents in rabbit coronary myocytes. Am J Physiol. 1994; 266:C1523–C1537.
9. Cook NS. Effect of some potassium channel blockers on contractile responses of the rabbit aorta. J Cardiovasc Pharmacol. 1989; 13:299–306.


10. Hara Y, Kitamura K, Kuriyama H. Actions of 4-aminopyridine on vascular smooth muscle tissues of the guinea-pig. Br J Pharmacol. 1980; 68:99–106.


11. Uchida Y, Nakamura F, Tomaru T, Sumino S, Kato A, Sugimoto T. Phasic contractions of canine and human coronary arteries induced by potassium channel blockers. Jpn Heart J. 1986; 27:727–740.


12. Ko EA, Han J, Jung ID, Park WS. Physiological roles of K+ channels in vascular smooth muscle cells. J Smooth Muscle Res. 2008; 44:65–81.


13. Bratz IN, Dick GM, Partridge LD, Kanagy NL. Reduced molecular expression of K+ channel proteins in vascular smooth muscle from rats made hypertensive with N{omega}-nitro-L-arginine. Am J Physiol Heart Circ Physiol. 2005; 289:H1277–H1283.
14. Wang Q, Curran ME, Splawski I, Burn TC, Millholland JM, Van-Raay TJ, Shen J, Timothy KW, Vincent GM, de Jager T, Schwartz PJ, Toubin JA, Moss AJ, Atkinson DL, Landes GM, Connors TD, Keating MT. Positional cloning of a novel potassium channel gene: KVLQT1 mutations cause cardiac arrhythmias. Nat Genet. 1996; 12:17–23.


15. Snyders DJ, Yeola SW. Determinants of antiarrhythmic drug action. Electrostatic and hydrophobic components of block of the human cardiac hKv1.5 channel. Circ Res. 1995; 77:575–583.
16. Lee HA, Hyun SA, Park SG, Kim KS, Kim SJ. Comparison of electrophysiological effects of calcium channel blockers on cardiac repolarization. Korean J Physiol Pharmacol. 2016; 20:119–127.


17. Kuriyama H, Kitamura K, Nabata H. Pharmacological and physiological significance of ion channels and factors that modulate them in vascular tissues. Pharmacol Rev. 1995; 47:387–573.
18. Hii JT, Wyse DG, Gillis AM, Cohen JM, Mitchell LB. Propafenone-induced torsade de pointes: cross-reactivity with quinidine. Pacing Clin Electrophysiol. 1991; 14:1568–1570.


19. Rehnqvist N, Ericsson CG, Eriksson S, Olsson G, Svensson G. Comparative investigation of the antiarrhythmic effect of propafenone (Rytmonorm) and lidocaine in patients with ventricular arrhythmias during acute myocardial infarction. Acta Med Scand. 1984; 216:525–530.


20. Slawsky MT, Castle NA. K+ channel blocking actions of flecainide compared with those of propafenone and quinidine in adult rat ventricular myocytes. J Pharmacol Exp Ther. 1994; 269:66–74.
21. Hoppe UC, Beuckelmann DJ. Modulation of the hyperpolarization-activated inward current (If) by antiarrhythmic agents in isolated human atrial myocytes. Naunyn Schmiedebergs Arch Pharmacol. 1998; 358:635–640.


22. Kim HS, Li H, Kim HW, Shin SE, Seo MS, An JR, Ha KS, Han ET, Hong SH, Choi IW, Choi G, Lee DS, Park WS. Escitalopram, a selective serotonin reuptake inhibitor, inhibits voltage-dependent K+ channels in coronary arterial smooth muscle cells. Korean J Physiol Pharmacol. 2017; 21:415–421.
23. Shin SE, Li H, Kim HS, Kim HW, Seo MS, Ha KS, Han ET, Hong SH, Firth AL, Choi IW, Bae YM, Park WS. Nortriptyline, a tricyclic antidepressant, inhibits voltage-dependent K+ channels in coronary arterial smooth muscle cells. Korean J Physiol Pharmacol. 2017; 21:225–232.
24. Li H, Kim HS, Kim HW, Shin SE, Jung WK, Ha KS, Han ET, Hong SH, Firth AL, Bae YM, Choi IW, Park WS. The class III anti-arrhythmic agent, amiodarone, inhibits voltage-dependent K+ channels in rabbit coronary arterial smooth muscle cells. Naunyn Schmiedebergs Arch Pharmacol. 2016; 389:713–721.
25. Xu C, Lu Y, Tang G, Wang R. Expression of voltage-dependent K+ channel genes in mesenteric artery smooth muscle cells. Am J Physiol. 1999; 277:G1055–G1063.
26. Jepps TA, Olesen SP, Greenwood IA. One man's side effect is another man's therapeutic opportunity: targeting Kv7 channels in smooth muscle disorders. Br J Pharmacol. 2013; 168:19–27.


27. Thorneloe KS, Chen TT, Kerr PM, Grier EF, Horowitz B, Cole WC, Walsh MP. Molecular composition of 4-aminopyridine-sensitive voltage-gated K+ channels of vascular smooth muscle. Circ Res. 2001; 89:1030–1037.
28. Li Q, Zhang R, Lü CL, Liu Y, Wang Z, Zhu DL. The role of subtypes of voltage-gated K+ channels in pulmonary vasoconstriction induced by 15-hydroeicosatetraenoic acid. Yao Xue Xue Bao. 2006; 41:412–417.
29. Cox RH, Fromme S. Functional expression profile of voltage-gated K+ channel subunits in rat small mesenteric arteries. Cell Biochem Biophys. 2016; 74:263–276.
30. Stott JB, Povstyan OV, Carr G, Barrese V, Greenwood IA. G-protein βγ subunits are positive regulators of Kv7.4 and native vascular Kv7 channel activity. Proc Natl Acad Sci U S A. 2015; 112:6497–6502.


31. Kang KW, Shim J, Ahn J, Lee DI, Kim J, Joung B, Choi KJ. 2018 Korean heart rhythm society guidelines for antiarrhythmic drug therapy in non-valvular atrial fibrillation. Korean J Med. 2018; 93:140–152.

