Abstract
Objective
Poly(lactide-co-glycolide) (PLGA) nanoparticles are promising materials for the development of new drug-releasing systems. The purpose of this study was to evaluate the in vivo retention time of materials loaded in nanoparticles as compared with that of the material alone by in vivo imaging in nude mice.
Materials and Methods
Mice (n = 20) were injected with 0.1 mL fluorescent material 1,1′-dioctadecyl-3,3,3′,3′ tetramethylindotricarbocyanine iodide (DiR)-loaded PLGA nanoparticles (200 nm) into the right paraspinal muscle, and the same volume of pure DiR solution was injected into the left paraspinal muscle. Fluorescence images were obtained using an in vivo optical imaging system. Fluorescent images were taken 1 day after the injection, and seven more images were taken at 1-week intervals. Image analysis was done with ImageJ program, and one region of interest was chosen manually, which corresponded to the highest signal-intensity area of fluorescence signal intensity.
Results
After 7 weeks, 12 mice showed a right-sided dominant signal, representing the DiR loaded PLGA nanoparticles; 5 mice showed a left-side dominant signal, representing the free DiR solution; and 3 mice showed no signal at all beginning 1 day after the injection. During the 7-week period, the mean signal intensities of the free DiR solution and DiR-loaded PLGA nanoparticles diverged gradually. On day 1, the mean signal intensity of free DiR solution was significantly higher than that of DiR-loaded PLGA (p < 0.001). Finally, by week 7, DiR-loaded PLGA express significantly high signal intensity compared with free DiR solution (p = 0.031).
As the population ages, chronic back pain is becoming one of the most frequently reported symptoms in the industrialized world. The estimated prevalence of nonspecific chronic low back pain in adults is 15%; however, this increases with age, reaching as high as 44% in individuals who are 70 years of age (12). Back pain can be caused by fracture, malignancy, inflammation, infection, deformity, or systemic diseases (12). However, chronic pain, defined as persistent pain that last 6 months after injury, is associated with chronic pathologic processes, and continually causes intermittent pain for months or years, is a complex problem that may not be amenable to routine pain control methods, and healing may never occur (3).
Because facet joints refer pain to adjacent structures, these types of joints are often a common cause of chronic back pain (45). Pain innervations are also present in other local soft tissue structures adjacent to the joint; thus, joint inflammation affects other local tissues (456). Facet joints can be blocked by intra-articular injections or by anesthetizing the medial branches of the dorsal rami, which innervate the target joint (67).
If the initial clinical and imaging findings are nonspecific or are insufficient for diagnosis, spinal pain intervention allows a functional assessment of the anatomic structures that are suspected to be sources of pain (89). Moreover, spinal pain intervention is needed as an adjunct to conservative management for patients with inoperable conditions, for postoperative pain and recovery, if oral or systemic drug therapy is discontinued because of adverse effects, or based on the presence of adjacent segment deterioration after spinal fusion (2).
Corticosteroids are commonly used in pain intervention and have both short-term and long-term effects. However, recurrent corticosteroid injection could reduce the degree of pain relief. Moreover, corticosteroids have severe systemic side effects, including infection, suppression of the pituitary-adrenal axis, hyperadrenocorticism, Cushing's syndrome, osteoporosis, avascular necrosis of the bone, steroid myopathy, epidural lipomatosis, weight gain, fluid retention, and hyperglycemia (10). Therefore, guidelines suggest limiting the frequency and interval of this type of pain intervention. The American Society of Interventional Pain Physicians recommends that facet joint injections be given at least 2–3 months apart assuming that more than 50% relief is obtained within 8 weeks.
Accordingly, there is an increased need to develop a new drug delivery system for improved local steroid retention. Kim et al. (11) used a rat model of spinal cord injury to compare the efficacy of controlled, nanoparticle-enabled local delivery of methylprednisolone to the injured spinal cord with systemic delivery and a single local injection of methylprednisolone without nanoparticles. Based on histological and behavioral data, they report that local, sustained delivery of methylprednisolone via nanoparticles is significantly more effective than systemic delivery.
The goal in our laboratory is to develop a new drug delivery system using poly(lactide-co-glycolide) (PLGA) nanoparticle-loaded triamcinolone for applications in the clinical setting. However, it is first necessary to confirm that the in vivo retention time of nanoparticle-loaded material is longer than that of the material alone. Therefore, the purpose of this study was to evaluate the in vivo retention time of materials loaded in nanoparticles as compared with that of the material alone by in vivo imaging in nude mice.
Positively charged PLGA nanoparticles doped with a fluorescent material, 1,1′-dioctadecyl-3,3,3′,3′ tetramethylindotricarbocyanine iodide (DiR), were synthesized by the College of Pharmacy, Chung-Ang University, Korea. In order to elucidate the effects of particle size, nanoparticles were made in three different sizes: 200 nm, 104 nm, and 105 nm. The particle size, polydispersity index, and zeta potential were measured with a Zetasizer Nano-ZS instrument (Malvern Instruments, Malvern, UK). The particle size distributions of 104 nm were measured using a Mastersizer 2000 instrument (Malvern Instruments). The particle size distributions of 105 nm were measured by sieving the particles through a series of meshes ranging from 80 µm to 180 µm. Nanoparticles containing DiR as the fluorescent probe were further characterized in terms of their particle size, zeta potential, and amount of DiR loading in aqueous medium (Table 1).
In order to determine the size of the particles (200 nm, 104 nm, and 105 nm) to be used in the main experiment, 3 nude mice were used in the pilot study. In the main study, the initial planned mice population was 30, which was calculated number by G*power program, when power (1-β error probability) = 0.8. However, 10 nude mice were died during experiment in the vivarium due to unknown cause. Consequentially, main study was performed with 20 nude mice (Fig. 1). A total of 33 hairless mice were obtained from Orient Bio (Seongnam, Korea). Mice were housed under conditions that included a controlled light cycle and controlled temperature (23 ± 1℃). Tap water and standard laboratory chow were available ad libitum. All animal experiments were performed in accordance with the Principles of Laboratory Animal Care (NIH publication number 85-23, revised 1996) and were approved by the Committee for Animal Experiments of Bundang Seoul National University Hospital (Seongnam, Korea).
For each animal, 0.1 mL DiR-loaded PLGA nanoparticles was injected into the right paraspinal muscles, and the same volume of pure DiR solution (50 µg/mL) was injected into the left paraspinal muscles. DiR solution was prepared by solubilizing the hydrophobic probe in 1% (w/v) sodium lauryl sulfate solution. Insulin syringes (31 G) were used for the injection, and all bilateral injections on the paraspinal back muscles were carried out by a single researcher.
A pilot study was performed in three nude mice with 200 nm, 104 nm, and 105 nm nanoparticles. Each mouse was injected different size of nanoparticles in order to determine whether there were differences in the in vivo characteristics of the nanoparticles according to particle size.
The main experiment was performed with 20 nude mice, and the smallest nanoparticles (200 nm) were used to evaluate the in vivo retention time of DiR-loaded PLGA nanoparticles as compared with that of pure DiR solution by in vivo imaging in nude mice.
Fluorescence images were obtained using an IVIS Imaging System (Lumina II; Caliper Life Sciences, Hopkinton, MA, USA). Fluorescent images were taken 1 day after the injection (day 1), and seven more images were taken at 1-week intervals (weeks 1–7). Fluorescence intensities were estimated using ImageJ, a public-domain Java image processing program, which can calculate area and pixel value statistics for user-defined selections. One region of interest (ROI) was chosen manually with the ImageJ program, which corresponded to the highest signal-intensity area of fluorescence signal intensity. Drawing of the ROI was done under the following guidelines: 1) should be an eclipse shape; 2) size of ROI on nanoparticle and control lesion in each mouse should be same; and 3) automatically calculated mean signal intensity was used in the result.
Statistical analysis was performed using PASW Statistics 19 software (IBM Corp., Armonk, NY, USA). Student's t test was used to compare the mean signal intensity values between free DiR solution and DiR-loaded PLGA nanoparticles in each week. Results with p values of less than 0.05 were considered significant.
Initially, three mice were injected with nanoparticles of three different sizes (200 nm, 104 nm, or 105 nm) loaded with the fluorescent material. The smallest particle used in this study, 200 nm gave a very rapid increase in fluorescence signal, reaching a maximum within 1 week (Fig. 2). The fluorescence signal remained consistent throughout the entire test period of 7 weeks. In contrast, the two larger particles (104 nm and 105 nm) showed a much slower increase in the fluorescence signal, reaching maxima at about week 6, near the end of the test period of 7 weeks, and the maximum values were lower than that of the 200 nm particles.
The retention profiles of DiR-loaded PLGA nanoparticles in the back muscles of nude mice were investigated by IVIS fluorescence imaging after intramuscular injection. In 14 of the 17 mice, free DiR solution showed stronger signal intensity than DiR-loaded PLGA nanoparticles on day 1 after injection (Table 2). After follow up of the 20 nude mice for 7 weeks, 12 mice showed a right-sided dominant signal (Table 2), representing the DiR-loaded PLGA nanoparticles; five mice showed a left-side dominant signal (Table 2), representing the free DiR solution; and three mice showed no signal at all beginning 1 day after the injection. These last three mice were excluded from further analysis owing to apparent injection failure. Figures 3 and 4 show IVIS images of mice #6 and #3 with the fluorescent signal of DiR-loaded PLGA nanoparticles and free DiR solution after intramuscular injection.
Notably, DiR-loaded PLGA nanoparticles showed significantly higher fluorescence intensity at the end of 7-week period (Table 3). During the 7-week period, the mean signal intensities of the free DiR solution and DiR-loaded PLGA nanoparticles diverged gradually. On day 1, the mean signal intensity of free DiR solution was 137.5 and that of DiR-loaded PLGA was 96.4, which showed significant differences (p < 0.001). For the next 6 weeks, the free DiR solution express gradual decreased signal intensity compared with the signal intensity of day 1, also, no statistical difference was observed in measuring free DiR solution against DiR-loaded PLGA nanoparticles in each week. Otherwise, mean signal intensity of DiR-loaded PLGA is relatively maintained during 7 weeks: week 1, 85.8; week 2, 98.6; week 3, 106.2; week 4, 101.3; week 5, 90.8; week 6, 97.7; and week 7, 94.1 (Table 3). Finally, by week 7, DiR-loaded PLGA express significantly high signal intensity compared with free DiR solution (p = 0.031).
Since the emergence of nanotechnologies, various fields have begun to utilize nanoparticles, including computer science, textile, cosmetic, and medical industries. Biodegradable nanoparticles are frequently used to improve bioavailability, retention times, and controlled delivery (12). Nanomedicines have been evaluated in clinical trials of different phases for potential applications in the treatment of cancer, acquire immunodeficiency syndrome, diabetes, tuberculosis, and malaria (13). In this study, we found that PLGA nanoparticle-loaded materials exhibited increased retention times in vivo, supporting the potential applications of PLGA nanoparticle-loaded materials in spinal intervention. Our experiment is a preliminary research and the ultimate goal is the development of a nanoparticle based steroid release system for epidural use. Since the increased concern about potential risks, such as the embolic infarction of particulate steroids, the use of non-particulate steroids increase in recent spine intervention, despite of its short duration of efficacy. It is necessary to develop a new type of injection material which can overcome the disadvantages of non-particulate steroids while having advantages of particulate steroids. Prior to evaluating the in vivo residue of the epidural injected nanoparticle-loaded steroid release system, a pilot study was needed to confirm whether the nanoparticle formulation could be remained in the body through actual animal image-based studies.
Nanoparticles are generally composed of positively charged polymers made through an emulsion and evaporation procedure. The nanoparticles provide a cationic surface shell structure with a neutral polymer core in which drug or fluorescence conjugates bind through ester bonds. Previous study has demonstrated prolonged intra-articular retention times through formation of cationic nanoparticles with a diffuse ionically crosslinked network of nanoparticles and endogenous hyaluronate in the knee joint (14). To the best of our knowledge, no studies have evaluated-intramuscular injection of nanoparticles at the paraspinal muscles.
Nanoparticle size could be a key factor affecting the differences in biodistribution. Leclerc et al. (15) reported that intramuscular injection of nanoparticles results in accumulation of nanoparticles in the circulatory system and testes as well as excretion of significant amounts of particles through the urine using mouse models. However, they confirmed that no morphological alterations occurred in the testis as a result of exposure to nanoparticles (15). In studies of the biodistribution of nanoparticles, researchers have found that smaller nanoparticles tend to reach the testes, whereas larger particles are unable to reach the testes (151617). De Jong et al. (17) intravenously injected rats with gold nanoparticles having diameters of 10, 50, 100, or 250 nm and found that the distribution of gold nanoparticles was size dependent, with the smallest particles showing widespread distribution to the blood, heart, lungs, liver, spleen, kidney, thymus, brain, and reproductive organs. Similarly, in our pilot study, we found that the smallest nanoparticles, having a size of 200 nm, showed the highest peak signal intensity within 1 week, which persisted throughout the test period. In contrast, 104 nm and 105 nm particles showed delayed signal release, with peak signal intensity observed at around 6 weeks after injection. Thus, further optimization of particle size may be needed for different applications. Prior studies evaluating the intra-articular injection of nanoparticles also emphasized that particle size as a key factor; particles smaller than 250 nm can freely escape from the joint cavity (18). Similarly, another study reported that nanoparticles with a mean diameter of 265 nm are phagocytosed in the synovium by macrophages that infiltrate through the synovial tissues, whereas particles measuring 26.5 µm in size are not phagocytosed. The phagocytosed nanoparticles are delivered to the deep underlying tissues; therefore, nanospheres are more suitable for delivery to inflamed synovial tissue than microspheres due to their ability to penetrate the synovium (19).
An interesting point is that, in the pilot study, 104 nm revealed low signal intensity compared with 105 nm particles during first 4 weeks. This finding is quite different from previous mentioned intraarticular or intramuscular studies (1518), which suggests biodistribution might be affected by various factors such as, vascularity and lymphatic drainage of injected site, or variation of pH.
The peak signal intensity of the 105 nm particles was lower than those of the 104 nm and 200-nm particles. Considering the minor variations of the signal intensity during seven weeks, delayed IVIS scan of 105 nm particles would be needed to provide a much higher signal intensity than the signal intensity observed at week 7. In this study, we used the 200-nm nanoparticles because we assumed that the rapid onset of the peak signal was more feasible when comparing with the signal intensity of the contralateral side.
Although prior studies (14181920) focused on the intra-articular local retention time, our results also demonstrated that the nanoparticle-loaded material was retained for a prolonged period in vivo. This in vivo examination of DiR-loaded PLGA nanoparticles confirmed that the nanoparticles exhibited significantly higher fluorescence signal intensity over the 7-week period after injection.
The inability to perform an in vitro study is the first limitation of our study. In the previous study, in vitro profile of DiR loaded nanoparticle produced an initial burst release in which approximately 30% of the molecules were released within a week and after that, there was continuous controlled release (20). This quick release could be arisen from the surface-associated probe and/or to the release of the probe encapsulated near to the particle surface (13). The PLGA nanoparticle used in our study was made in the same way as that used in the previous paper (20), so in vitro profile of our nanoparticle is also predicted that there would be a controlled release property. Further, we plan additional research, including in vitro releasing profile of nanoparticles. Second, intramuscular injection could be a limitation in direct clinical application of nanoparticles in slow releasing drug delivery system. Initially, we tried to make facet joint injection model of rat and mouse, but it was not feasible. The epidural injection in chronic back pain is actually a steroid injection in the periarticular space, so we planned paraspinal muscle injection aiming injection around vertebra. Further study can try a facet joint injection in an animal with larger than a certain size such as a dog or pig. Third, there is a possibility that the statistical power is decreased due to the many number of animals died in the middle of the study. Fourth, if additional IVIS scans were taken after 8 weeks, the DiR loaded nanoparticle would have shown consistently high signal intensity. As there was a statistically significant difference at week 7 between the signal intensity of DiR loaded nanoparticle and free DiR solution, we did not plan to extend the experiment period. However, this can be a limiting factor for a more stable result. Additionally, other therapeutic material, such as steroid-loaded nanoparticles, could perform differently from our DiR-loaded nanoparticles; therefore further studies are needed.
In summary, the results of the current study suggested that therapeutic agents that bind to PLGA nanoparticles may exhibit prolonged retention times. Further studies are needed to optimize particle size, evaluate drug-loading capacity, and perform in vivo retention assessments in animal models to more closely mimic the clinical setting.
References
1. Manchikanti L, Singh V, Pampati V, Beyer CD, Damron KS. Evaluation of the prevalence of facet joint pain in chronic thoracic pain. Pain Physician. 2002; 5:354–359. PMID: 16886012.


2. Fritz J, Niemeyer T, Clasen S, Wiskirchen J, Tepe G, Kastler B, et al. Management of chronic low back pain: rationales, principles, and targets of imaging-guided spinal injections. Radiographics. 2007; 27:1751–1771. PMID: 18025516.


3. Boswell MV, Trescot AM, Datta S, Schultz DM, Hansen HC, Abdi S, et al. Interventional techniques: evidence-based practice guidelines in the management of chronic spinal pain. Pain Physician. 2007; 10:7–111. PMID: 17256025.
4. Fukui S, Ohseto K, Shiotani M, Ohno K, Karasawa H, Naganuma Y, et al. Referred pain distribution of the cervical zygapophyseal joints and cervical dorsal rami. Pain. 1996; 68:79–83. PMID: 9252002.


5. Fukui S, Ohseto K, Shiotani M, Ohno K, Karasawa H, Naganuma Y. Distribution of referred pain from the lumbar zygapophyseal joints and dorsal rami. Clin J Pain. 1997; 13:303–307. PMID: 9430810.


6. Boswell MV, Colson JD, Spillane WF. Therapeutic facet joint interventions in chronic spinal pain: a systematic review of effectiveness and complications. Pain Physician. 2005; 8:101–114. PMID: 16850048.
7. Boswell MV, Colson JD, Sehgal N, Dunbar EE, Epter R. A systematic review of therapeutic facet joint interventions in chronic spinal pain. Pain Physician. 2007; 10:229–253. PMID: 17256032.
8. Jarvik JG, Deyo RA. Diagnostic evaluation of low back pain with emphasis on imaging. Ann Intern Med. 2002; 137:586–597. PMID: 12353946.


9. Bogduk N. International Spinal Injection Society guidelines for the performance of spinal injection procedures. Part 1: zygapophysial joint blocks. Clin J Pain. 1997; 13:285–302. PMID: 9430809.
10. Manchikanti L. Role of neuraxial steroids in interventional pain management. Pain Physician. 2002; 5:182–199. PMID: 16902669.


11. Kim YT, Caldwell JM, Bellamkonda RV. Nanoparticle-mediated local delivery of Methylprednisolone after spinal cord injury. Biomaterials. 2009; 30:2582–2590. PMID: 19185913.


12. Alexis F, Pridgen E, Molnar LK, Farokhzad OC. Factors affecting the clearance and biodistribution of polymeric nanoparticles. Mol Pharm. 2008; 5:505–515. PMID: 18672949.


13. Kumari A, Yadav SK, Yadav SC. Biodegradable polymeric nanoparticles based drug delivery systems. Colloids Surf B Biointerfaces. 2010; 75:1–18. PMID: 19782542.


14. Morgen M, Tung D, Boras B, Miller W, Malfait AM, Tortorella M. Nanoparticles for improved local retention after intra-articular injection into the knee joint. Pharm Res. 2013; 30:257–268. PMID: 22996566.


15. Leclerc L, Klein JP, Forest V, Boudard D, Martini M, Pourchez J, et al. Testicular biodistribution of silica-gold nanoparticles after intramuscular injection in mice. Biomed Microdevices. 2015; 17:66. PMID: 26044201.


16. Morishita Y, Yoshioka Y, Satoh H, Nojiri N, Nagano K, Abe Y, et al. Distribution and histologic effects of intravenously administered amorphous nanosilica particles in the testes of mice. Biochem Biophys Res Commun. 2012; 420:297–301. PMID: 22417826.


17. De Jong WH, Hagens WI, Krystek P, Burger MC, Sips AJ, Geertsma RE. Particle size-dependent organ distribution of gold nanoparticles after intravenous administration. Biomaterials. 2008; 29:1912–1919. PMID: 18242692.


18. Horisawa E, Kubota K, Tuboi I, Sato K, Yamamoto H, Takeuchi H, et al. Size-dependency of DL-lactide/glycolide copolymer particulates for intra-articular delivery system on phagocytosis in rat synovium. Pharm Res. 2002; 19:132–139. PMID: 11883639.
19. Levick JR. A method for estimating macromolecular reflection by human synovium, using measurements of intra-articular half lives. Ann Rheum Dis. 1998; 57:339–344. PMID: 9771207.


20. Kim SR, Ho MJ, Lee E, Lee JW, Choi YW, Kang MJ. Cationic PLGA/Eudragit RL nanoparticles for increasing retention time in synovial cavity after intra-articular injection in knee joint. Int J Nanomedicine. 2015; 10:5263–5271. PMID: 26345227.
Fig. 3
In vivo fluorescence images of mouse 6.
Left paraspinal muscle, pure DiR solution; right paraspinal muscle, DiR-loaded PLGA nanoparticles. Signal intensity of pure DiR solution (dot lines), injected at left paraspinal muscle, shows bright yellow signal intensity on day 1, maintained until week 3, and slightly decreased from week 4. On other hand, DiR-loaded PLGA nanoparticles (arrows), injected at right paraspinal muscle, shows gradual signal increase. At end of study on week 7, DiR-loaded PLGA nanoparticles of right paraspinal muscle express bright yellow spot, whereas pure DiR solution in contralateral side shows reddish color. DiR = 1,1′-dioctadecyl-3,3,3′,3′ tetramethylindotricarbocyanine iodide, L = left, PLGA = poly(lactide-co-glycolide), R = right

Fig. 4
In vivo fluorescence images of mouse 3.
Left paraspinal muscle, pure DiR solution; right paraspinal muscle, DiR-loaded PLGA nanoparticles. On day 1, both sides show similar bright yellow signal intensity. Signal intensity of pure DiR solution (dot lines), injected at left paraspinal muscle, shows gradual signal decrease. DiR-loaded PLGA nanoparticles (arrows), injected at right paraspinal muscle, express bright yellow signal during 7 weeks. At end of study, DiR-loaded PLGA nanoparticles of right paraspinal muscle still express bright yellow spot, whereas pure DiR solution in contralateral side shows no measurable signal intensity.

Table 1
Physicochemical Characteristics of DiR-Loaded PLGA Nanoparticles
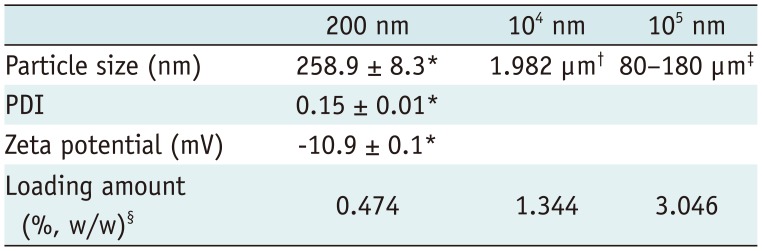
200 nm | 104 nm | 105 nm | |
---|---|---|---|
Particle size (nm) | 258.9 ± 8.3* | 1.982 µm† | 80–180 µm‡ |
PDI | 0.15 ± 0.01* | ||
Zeta potential (mV) | −10.9 ± 0.1* | ||
Loading amount (%, w/w)§ | 0.474 | 1.344 | 3.046 |
Values represent means ± SDs. *Particle size, PDI, and zeta potential measured with Zetasizer Nano-ZS (Marlvern Instrument), †Particle size distribution of 104 nm was measured using Mastersizer 2000 (Marlvern Instrument), ‡Particle size distribution of 105 nm was measured by sieving through series of meshes ranging from 80 µm to 180 µm, §Loading amount (%, w/w) = (weight of encapsulated DiR) / (total weight of PLGA particles) × 100. DiR = 1,1′-dioctadecyl-3,3,3′,3′ tetramethylindotricarbocyanine iodide, PDI = polydispersity index, PLGA = poly(lactide-co-glycolide), SD = standard deviation
Table 2
Signal Intensity on Day Just after Injection and at End of Week 7
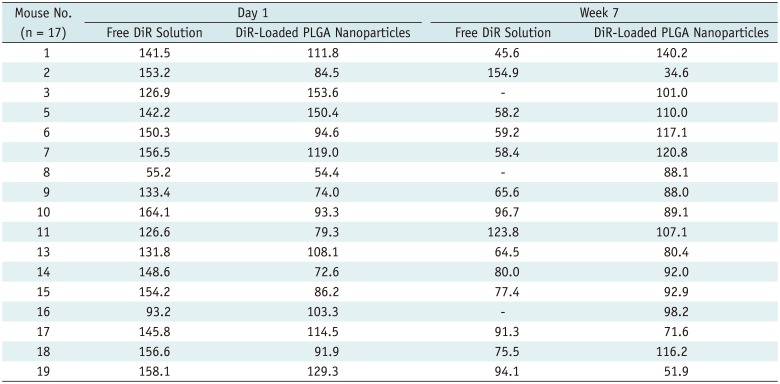
Table 3
Comparison of Mean Signal Intensity Values between Free DiR Solution and DiR-Loaded PLGA Nanoparticles during 7 Weeks in 17 Mice
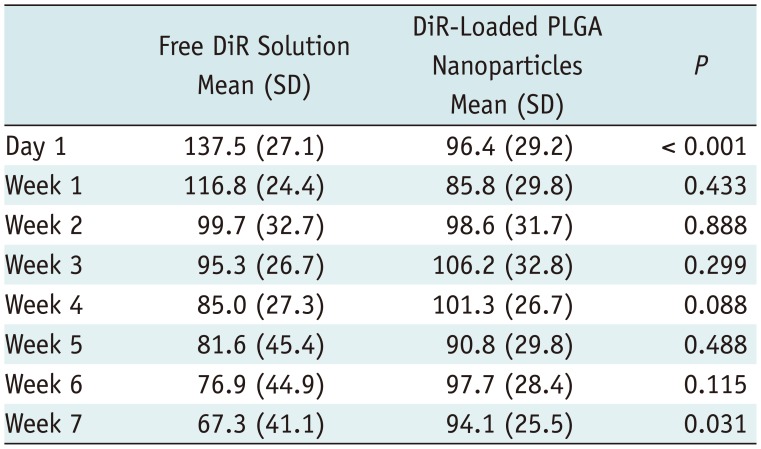