Abstract
Accurate identification of the epileptogenic zone is an important prerequisite in presurgical evaluation of refractory epilepsy since it affects seizure-free outcomes. Apart from structural magnetic resonance imaging (sMRI), delineation has been traditionally done with electroencephalography and nuclear imaging modalities. Arterial spin labelling (ASL) sequence is a non-contrast magnetic resonance perfusion technique capable of providing similar information. Similar to single-photon emission computed tomography, its utility in epilepsy is based on alterations in perfusion linked to seizure activity by neurovascular coupling. In this article, we discuss complementary value that ASL can provide in the evaluation and characterization of some basic substrates underlying epilepsy. We also discuss the role that ASL may play in sMRI negative epilepsy and acute scenarios such as status epilepticus.
Symptomatic epilepsy is defined as epilepsy having gross anatomic or pathologic abnormalities secondary to anacquired or genetic cause (1). Its natural course and response to medical treatment are determined by its etiology. Approximately 25% of symptomatic epilepsy cases are refractory (2). In such cases, accurate localization of the epileptogenic zone (EZ) is one of the strongest prognostic factors since it allows complete surgical resection and effective seizure control (3). Currently, localization is achieved by a combination of seizure semiology and electroencephalography (EEG) along with structural magnetic resonance imaging (sMRI). In cases with negative sMRI or discordant findings, interictal 18-flurodeoxyglucose positron emission tomography (PET) and ictal single-photon emission computed tomography (SPECT) can be used for localization. However, these modalities involve ionizing radiation with limited resolution. Although invasive EEG is highly accurate, it has a limited sampling area (4). A more circumscribed localization with non-invasive imaging can help guide the placement of these electrodes (5).
Arterial spin labelling (ASL)-based magnetic resonance (MR) perfusion is a technique that has potential to be used as a non-invasive and non-irradiating supplementary method of localization. In this article, we review clinical insights and advantages gained from its use in the evaluation of epilepsy.
Arterial spin labelling uses an inversion pulse to tag flowing blood in the neck. Following a short post-labelling delay (PLD) to allow for intracranial transit, these ‘labelled’ spins are imaged during exchange into the brain parenchyma. Subtracting a paired set of ‘labelled’ and unlabelled ‘control’ images will yield parametric maps of absolute cerebral blood flow (CBF) that may be used for qualitative and quantitative evaluation. In effect, ASL uses blood as an endogenous contrast material, making it non-invasive and repeatable. Thus, it has significant advantages, particularly for pediatric population (6).
Scans are performed on a 3T GE Discovery 750w scanner (GE Healthcare, Milwaukee, USA) using a three dimensional-pseudocontinuous ASL sequenceprior to contrast administration per recommendations by the International Society for Magnetic Resonance in Medicine (5). Post-processing includes CBF quantification and fusion with relevant sMRI sequences.
Two important concepts are necessary to understand the aim and utility of ASL in epilepsy: the EZ and the principle of neurovascular coupling.
The part of the cortex from which seizures originate is referred to as the ictal onset zone (IOZ). Some lesions such as arteriovenous malformations (AVMs) and tumors have a perilesional EZ that does not necessarily overlap with the IOZ. It is capable of generating seizures by itself even after removing the inciting lesion. Spread of epileptic activity to adjacent areas will manifest as semiology of the seizure. This is designated as the symptomatogenic zone. A functional deficit zone (FDZ) corresponds to areas with altered interictal function while the irritative zone generates interictal spikes that are secondary to inhibitory neural networks (Fig. 1). EZ represents the minimum amount of tissue that must be surgically resected or disconnected. Its accurate delineation is the holy grail of presurgical evaluation since it impacts post-operative seizure freedom and neurocognitive outcomes (5789). Evidence has emerged that FDZ identified by neuropsychological evaluation or regions of PET hypometabolism may expand horizons of EZ, particularly in temporal lobe epilepsy (TLE), thus requiring additional resection (91011). EZ is thus a theoretical construct that can only be estimated based on combined evidence from seizure semiology, electrophysiological tests, and imaging. Alterations in perfusion as reflected on ASL may occur not only in EZ, but also in FDZ and symptomatogenic zones. ASL has a reasonable overall accuracy of 70% in localizing the seizure focus, which is lower than the overall accuracy of 95% by electroclinical localization (12). Thus, interpretation of ASL in isolation is limited. Electroclinical evidence must be considered to reach a more accurate estimation of EZ location.
Neurovascular coupling is the phenomenon that links transient neural activity to a corresponding increase in regional CBF. This is an active process. It is not merely dependent on an oxygen debt as previously thought. Changes in neuronal activity can modulate vascular smooth muscle tone via neuronal synaptic activity and glial release of vasoactive mediators occurring at the level of neurovascular unit (13). Although highly controversial, neurovascular coupling has been shown to be largely intact within the EZ (141516). This forms the basis of perfusion techniques such as ASL and SPECT in detecting alterations of blood flow related to abnormal synaptic activity. Most epileptogenic foci are hypoperfused during the interictal period and become hyperperfused during seizures. This indirect information must always be correlated for concordance with the original electroclinical hypothesis since this may encompass the IOZ and potentially FDZ (17).
Positron emission tomography has been found to have higher level of concordance with electroclinical localization in mesial TLE (MTLE) (78%) than that in extratemporal epilepsy (28.6%) (18). Data from SPECT studies have shown that this sensitivity is increased when patient is additionally imaged in the peri-ictal period compared to in the interictal period alone (19). Hence, a significant advantage of ASL over PET is that it can show both peri-ictal and interictal abnormalities. This implies that, for a useful evaluation of ASL, it is imperative to know if the patient is imaged in the peri-ictal or interictal period based on clinical history or EEG findings and compare focal abnormalities on ASL to seizure semiology and expected hemodynamics of the lesion.
We will describe the utility of ASL in the evaluation of five basic substrates for epilepsy: 1) mesial temporal sclerosis, 2) malformations of cortical development (MCDs), 3) neoplasms, 4) vascular malformations, and 5) infections, inflammations, and gliosis. In addition, we will describe the application of ASL in sMRI-negative epilepsy. Where studies on ASL specific to the entity are lacking, we will describe comparable modalities such as PET and dynamic susceptibility contrast (DSC) imaging.
Hippocampal sclerosis is the most common cause of intractable epilepsy (2). Approximately 20–30% of patients with MTLE have no sMRI identifiable abnormality (20). While visual inspection of ASL CBF maps may identify obvious areas of altered perfusion (Fig. 2), quantitative methods are needed for cases with ambiguous lateralization. Mean CBF values derived by regions of interest over the right (R) and left (L) mesial temporal regions may be used to calculate Asymmetry Index (AI) using the following formula: AI = 100 × (L − R) / (R − L), with negative values indicating left lateralization (21). However, caution is needed since normal subjects may show physiological left temporal lobe hyperperfusion. Seizure frequency and severity inversely are correlated with ipsilateral hippocampal CBF, but not with the magnitude of AI possibly due to secondary involvement of contralateral temporal lobe (2223). Since these changes occur in sMRI-negative cases as well, ASL can improve the overall sensitivity of MRI. It has shown a good concordance with both PET and electrical source imaging (242526). Another important caveat is peri-ictal hippocampal changes in conditions such as status epilepticus that may occur regardless of the site of ictal onset. Interval imaging may be necessary to look for reversibility or evolution into sclerosis (2728).
Malformations of cortical development comprise between 10–50% of pediatric and 4–25% of adult surgical epilepsy patients (29). Focal cortical dysplasias (FCDs) are probably the most common cause of intractable extratemporal epilepsy (30). On nuclear imaging, FCDs commonly show interictal hypometabolism and peri-ictal hypermetabolism. These areas typically appear larger than sMRI-visible lesion (Fig. 3) (31). While this has conventionally been attributed to altered synaptic/epileptic activity, Wintermark et al. (32) have suggested that these findings might be related to higher microvessel density measured by immunohistochemical staining of CD34 positive endothelial cells on surgical resection specimens. They also found significantly higher vascular density in the adjacent normal appearing cortex and hypothesized that interictally hypervascular FCDs might require larger resection margin (32). ASL is also a viable substitute for nuclear imaging to localize the offending tuber in tuberous sclerosis (33).
Hemimegalencephaly is a prime example of pathology in which perfusion and metabolism may be uncoupled. Involved areas may show variable metabolic activities on PET with a paradoxically opposite perfusion pattern on SPECT that suggests a role of either vascular proliferative abnormalities or subclinical seizures (34). ASL has shown similar variability in perfusion of these lesions (Fig. 4) (3536).
Polymicrogyric cortex shows variable patterns of metabolic activity compared to normal cortex on PET (37). Band heterotopia may show similar or higher metabolic activity compared to overlying cortex appearing as a ‘double cortex’ similar to structural imaging. In both these entities, altered perfusion might be related to synaptic activity of neurons regardless of whether it results in clinical seizures (3839). ASL shows perfusion patterns concordant with PET (Fig. 5). ASL also allows ruling out of contralateral perfusion anomalies related to microstructural or network alterations since these anomalies could potentially impact post-surgical outcomes (3140).
Tumors associated with drug resistant epilepsy for a duration of usually two years or longer are termed long-term epilepsy associated tumors, with gangliogliomas and dysembryoplastic neuroepithelial tumors being the commonest (41). Early resection is preferred for both seizure control and tumor remission (42). The utility of ASL in predicting tumor grade has been well established (43). However, when the primary goal of surgery is seizure control, EZ, not just the tumor, should be resected. It has been shown that EEG and ictal SPECT are useful in delineating EZ around tumors (44). Peri-ictal ASL has similar potential. It may reduce the need for a separate study. Alterations of perfusion may also represent ictal activity propagation to a symptomatogenic zone (Fig. 6).
Seizures in vascular malformations are caused by neuronal toxicity and gliosis secondary to hemosiderin deposition during overt or subclinical hemorrhage. Chronic venous congestion, impaired peri-nidal cerebrovascular reserve, and steal phenomena are additional factors in the epileptogenicity of AVMs (45).
Arterial spin labelling allows characterization of both vascular malformation and its secondary effects on the brain parenchyma. Venous signal on ASL is a sensitive marker of shunting in occult malformations (46). Using a single compartment model, semi-quantitiative estimation of shunt fraction can be calculated. Multi-PLD ASL allows estimation of transit times. It is useful for monitoring patients after shunt reduction interventions or radiosurgery (47). Peri-nidal hyperperfusion and steal phenomena can also be identified and quantified (Fig. 7). Areas of peri-ictal hyperperfusion distant from the AVM nidus have been found to be consistent with secondary EZs (48).
Epileptogenic cavernomas may demonstrate peri-ictal hyperperfusion of the adjacent cortex (49). Developmental venous anomalies may also be associated with epilepsy, even when they are isolated (50). Although variable peri-lesional parenchymal perfusion changes may be seen, hyperperfusion must be interpreted with caution since it might be related to micro-shunting within the parenchymal microvasculature, not necessarily a peri-ictal change (51). In Sturge-Weber syndrome, DSC perfusion studies have demonstrated that white matter perfusion is increased in early stages with progressive hypoperfusion with increasing severity and duration of epilepsy (52).
Rasmussen's syndrome is the prototypical T-cell mediated pediatric immune disorder characterized by refractory focal seizures and development of progressive unilateral neurological deficits (53). PET and ASL imaging have shown areas of hypermetabolism/perfusion consistent with ictal activity on EEG. However, dissociated area of hyperperfusion might be related to ongoing inflammation. It can be used as a marker of disease activity (Fig. 8) (5455).
Other humorally-mediated autoimmune epilepsies clinically present with a syndrome of subacute limbic or neocortical encephalitis (56) characterized by variable limbic or extralimbic sMRI abnormalities (57). ASL may show peri-ictal hyperperfusion. However, changes related to inflammation may precede sMRI findings. They can be used to monitor response to therapy (5859).
Seizures can occur during the acute phase of infection or as its sequelae. Epileptogenicity is multifactorial and dependent on pathogen virulence, lesion type, degree of cortical involvement, and host inflammatory response (60). In herpes simplex encephalitis, ASL shows hyperperfusion consistent with inflammation which may help differentiate other hypoperfused lesions such as diffuse astrocytomas. In meningitis, gyral hyperperfusion,and less commonly, hypoperfusion, may be seen (Fig. 9). Abscesses show low perfusion along their rims, differentiating them from necrotic glioblastomas (61). Whole-brain CBF has been found to be reduced in human immunodeficiency virus-related encephalopathy. However, in progressive multifocal leukoencephalopathy, hyperperfusion is associated with disease progression and inversely related to the incidence of immune reconstitution inflammatory syndrome (6263). Thus, ASL can lead to better characterization of pathogen-host relationship. It might be used to non-invasively monitor response to therapy.
Gliosis is identified by non-specific neuronal loss and reactive astrogliosis. The most common causes encountered in epilepsy are those secondary to perinatal insults, infarctions, trauma, and demyelination (29). Surgical outcomes are inferior in this subgroup as pathologies are poorly circumscribed, often involving eloquent cortex that constrains complete resections (64). Most encephalomalacic lesions are characterized by interictal hypoperfusion on ASL (65). Hypoperfusion extending beyond the electroclinical EZ or sMRI abnormal areas can adversely impact outcomes. Peri-ictal hyperperfusion of the overlying cortex in post-stroke seizures and in Wilson's disease (Fig. 10) has also been reported (666768).
An estimated 20–40% of surgical epilepsy patients have no identifiable causative lesion on sMRI. This adversely affects prognosis and presents difficulties for surgical resection (69). The most common underlying pathologies identified are FCDs, gliosis, hamartomas, and hippocampal sclerosis, with the rest being negative for any histopathological abnormality (7071). Invasive EEG monitoring before surgical resection can be aided by prior non-invasive lateralization or localization. PET has shown a high concordance of lateralization with intracranial EEG (44.3–100%), but a more variable localization value (11–69% concordance) (72). PET positivity itself is a good prognosticator for post-surgical outcomes (20). ASL has shown excellent and high concordances with PET in lateralization and localization of EZ. It is a promising utility in the evaluation of MR-negative epilepsies (Fig. 11) (73).
Failure to terminate seizures can lead to permanent neurological damage termed status epilepticus (or epilepsia partialis continua for partial seizures). A less easily recognized ‘non-convulsive’ status may also occur (74). sMRI may disclose a range of findings, including gyral swelling and secondary hippocampal or thalamic involvement with variable diffusion restriction, persistence of which indicates poorer outcomes. ASL demonstrates focal or diffuse hyperemia with high sensitivity and concordance with EEG (49). Distribution of abnormalities may encompass the EZ as well as areas of seizure propagation (Fig. 12) (4968). ASL also allows serial follow-up and prognostication (7475).
Accurate identification of the EZ is crucial in presurgical evaluation of epilepsy since it influences prognosis. ASL is a non-contrast MR perfusion technique that demonstrates alterations in perfusion related to abnormal synaptic activity, akin to nuclear imaging. This not only allows localization of the epileptogenic focus, but also provides functional characterization of abnormalities identified by sMRI. ASL is a useful supplementary investigation. It has potential to make MRI a one-stop-shop in epilepsy evaluation.
Figures and Tables
Fig. 1
Various zones around epileptogenic lesion.
Successful surgical outcome is dependent on resection of EZ. EZ = epileptogenic zone
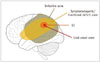
Fig. 2
Hippocampal sclerosis.
Peri-ictal imaging in 14-year-old female child who presented with new-onset hypomotor complex partial seizures showing swelling and T2 hyperintensity of right hippocampus (arrow, A) with hyperperfusion on ASL fusion images (arrow, B). Unlike this particular case, chronic hippocampal sclerosis with secondary atrophy commonly show hypoperfusion on ASL. ASL = arterial spin lebelling
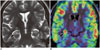
Fig. 3
Focal cortical dysplasia.
Interictal imaging in 24-year-old female with occasional breakthrough seizures showing right frontal Type II FCD on FLAIR (arrow, A) with hypoperfusion on corresponding ASL fusion images (arrow, B). In another case of 11-year-old female child with refractory extratemporal complex partial seizures, peri-ictal imaging showing right parietal FCD on T1 SPGR (arrow, C) and focal hyperperfusion on ASL grey-scale source images (arrow, D) consistent with active seizure focus. FCD = focal cortical dysplasia, FLAIR = fluid attenuation inversion recovery, SPGR = spoiled gradient recalled acquisition
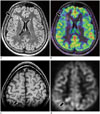
Fig. 4
Hemimegalencephaly.
Note subtle enlargement of left cerebral hemisphere on FLAIR (most obvious in regions between arrows in A) in 16-year-old male with global developmental delay and refractory seizures. Interictal ASL fusion images (B) showing areas of cortical hypoperfusion that allow more clear delineation of extent of involvement (between arrows in B).
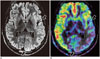
Fig. 5
Polymicrogyria.
Left perisylvian polymicrogyria seen on T1 SPGR (arrows, A) in 25-year-old male with extratemporal complex partial seizures. Focal areas of hyperperfusion are seen on ASL fusion images of polymicrogyric cortex (arrows, B) compared to normal perfusion pattern of contralateral side (C) in this peri-ictal scan.
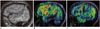
Fig. 6
Dysembryoplastic neuroepithelial tumor.
T2-weighted images (A) showing dysembryoplastic neuroepithelial tumor involving right peri-Rolandic region in 24-year-old male. While lesion per se is hypoperfused, adjacent hyperperfusion involves lateral right sensorimotor cortex (arrow, B) on this peri-ictal scan despite sMRI showing lack of perilesional changes, concordant with presentation of simple partial seizures of left hand despite lesion was located in foot region. sMRI = structural magnetic resonance imaging
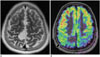
Fig. 7
Arteriovenous malformation.
Unruptured AVM involving left frontal lobe as seen on MR angiography (black arrow, A) in 34-year-old male with secondary generalized seizures. Shunting as depicted by intravascular signal within AVM nidus (black arrow, B) and draining superior sagittal sinus (black arrow, C) is seen on ASL color cerebral blood flow maps. Note hypoperfusion involving rest of left middle cerebral artery territory (best seen at white arrows in B, C). Catheter angiography confirmed high flow shunting with attenuation of ipsilateral parenchymal capillary blush (D) compared to contralateral normal side (E). Steal phenomena like these are known causes of seizures in AVMs. AVM = arteriovenous malformation
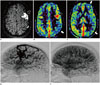
Fig. 8
Rasmussen's syndrome.
Left hemispheric involvement in 15-year-old girl post-callosotomy. FLAIR (A), shows burnt-out disease in atrophied left frontal region (black arrow, A) which shows corresponding low perfusion on ASL (black arrow, B). Persistent marked hyperperfusion along left parieto-occipital cortex (white arrows, B) despite seizure-free post-surgical outcome suggests concomitant ongoing inflammation.
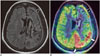
Fig. 9
Tubercular meningitis.
Biopsy-proven case in 44-year-old female with secondary generalized seizures and depressed sensorium. Enhancing dura-based granulomatous lesion is seen on T1-weighetd image (black arrow, A) with adjacent cortex showing significant hypoperfusion on ASL (white arrows, B) in this peri-ictal scan.
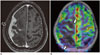
Fig. 10
Sequelae of periventricular hemorrhagic infarction.
12-year-old boy with daily seizures and stormy perinatal history. Ulegyria diffusely involves entire right cerebral hemisphere on T1 (A). Peri-ictal ASL fusion images (B) show significant hyperperfusion involving posterior regions (arrows, B), allowing localization of seizure focus concordant with electroencephalography findings.
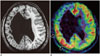
Fig. 11
sMRI negative temporal epilepsy.
15-year-old boy with temporal neocortical epilepsy. Hippocampi appeared normal on T2-weighted image (A). Right temporal hypoperfusion on ASL (arrows, B) suggested right temporal EZ. Confirmatory positron emission tomography showing excellent concordance with ASL (arrows, C).
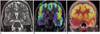
Fig. 12
Epilepsia partialis continua.
13-year-old boy presented with continuous right hemispheric partial seizures. FLAIR showed right frontal FCD (arrow, A) lacking significant changes in rest of ipsilateral hemisphere (not shown). Ictal ASL showed intense hyperperfusion of FCD along with secondary hyperperfusion in rest of ipsilateral cerebrum (B, C). This likely represents spread of seizure activity to symptomatogenic zone.
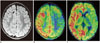
References
2. Koepp MJ, Woermann FG. Imaging structure and function in refractory focal epilepsy. Lancet Neurol. 2005; 4:42–53.


3. Woermann FG, Vollmar C. Clinical MRI in children and adults with focal epilepsy: a critical review. Epilepsy Behav. 2009; 15:40–49.


4. Tao JX, Baldwin M, Hawes-Ebersole S, Ebersole JS. Cortical substrates of scalp EEG epileptiform discharges. J Clin Neurophysiol. 2007; 24:96–100.


5. Shorvon S, Perucca E, Engel J Jr. The treatment of epilepsy. 4th ed. Hoboken, NJ: Wiley-Blackwell;2015. p. 714–715.
6. Deibler AR, Pollock JM, Kraft RA, Tan H, Burdette JH, Maldjian JA. Arterial spin-labeling in routine clinical practice, part 1: technique and artifacts. AJNR Am J Neuroradiol. 2008; 29:1228–1234.


7. Blumenfeld H, McNally KA, Vanderhill SD, Paige AL, Chung R, Davis K, et al. Positive and negative network correlations in temporal lobe epilepsy. Cereb Cortex. 2004; 14:892–902.


8. Spencer SS. Neural networks in human epilepsy: evidence of and implications for treatment. Epilepsia. 2002; 43:219–227.


9. Englot DJ, Raygor KP, Molinaro AM, Garcia PA, Knowlton RC, Auguste KI, et al. Factors associated with failed focal neocortical epilepsy surgery. Neurosurgery. 2014; 75:648–656. discussion 655; quiz 656.


10. Chassoux F, Artiges E, Semah F, Laurent A, Landré E, Turak B, et al. 18F-FDG-PET patterns of surgical success and failure in mesial temporal lobe epilepsy. Neurology. 2017; 88:1045–1053.
11. Theodore WH. Presurgical focus localization in epilepsy: PET and SPECT. Semin Nucl Med. 2017; 47:44–53.


12. Kim BS, Lee ST, Yun TJ, Lee SK, Paeng JC, Jun J, et al. Capability of arterial spin labeling MR imaging in localizing seizure focus in clinical seizure activity. Eur J Radiol. 2016; 85:1295–1303.


13. Huneau C, Benali H, Chabriat H. Investigating human neurovascular coupling using functional neuroimaging: a critical review of dynamic models. Front Neurosci. 2015; 9:467.


14. Gaillard WD, Fazilat S, White S, Malow B, Sato S, Reeves P, et al. Interictal metabolism and blood flow are uncoupled in temporal lobe cortex of patients with complex partial epilepsy. Neurology. 1995; 45:1841–1847.


15. Hamandi K, Laufs H, Nöth U, Carmichael DW, Duncan JS, Lemieux L. BOLD and perfusion changes during epileptic generalised spike wave activity. Neuroimage. 2008; 39:608–618.


16. Stefanovic B, Warnking JM, Kobayashi E, Bagshaw AP, Hawco C, Dubeau F, et al. Hemodynamic and metabolic responses to activation, deactivation and epileptic discharges. Neuroimage. 2005; 28:205–215.


17. Pizzini FB, Farace P, Manganotti P, Zoccatelli G, Bongiovanni LG, Golay X, et al. Cerebral perfusion alterations in epileptic patients during peri-ictal and post-ictal phase: PASL vs DSC-MRI. Magn Reson Imaging. 2013; 31:1001–1005.


18. Menon RN, Radhakrishnan A, Parameswaran R, Thomas B, Kesavadas C, Abraham M, et al. Does F-18 FDG-PET substantially alter the surgical decision-making in drug-resistant partial epilepsy? Epilepsy Behav. 2015; 51:133–139.


19. Madan N, Grant PE. New directions in clinical imaging of cortical dysplasias. Epilepsia. 2009; 50:Suppl 9. 9–18.


20. Jones AL, Cascino GD. Evidence on use of neuroimaging for surgical treatment of temporal lobe epilepsy: a systematic review. JAMA Neurol. 2016; 73:464–470.
21. Gaillard WD, Zeffiro T, Fazilat S, DeCarli C, Theodore WH. Effect of valproate on cerebral metabolism and blood flow: an 18F-2-deoxyglucose and 15O water positron emission tomography study. Epilepsia. 1996; 37:515–521.
22. Guo X, Xu S, Wang G, Zhang Y, Guo L, Zhao B. Asymmetry of cerebral blood flow measured with three-dimensional pseudocontinuous arterial spin-labeling mr imaging in temporal lobe epilepsy with and without mesial temporal sclerosis. J Magn Reson Imaging. 2015; 42:1386–1397.


23. Wolf RL, Alsop DC, Levy-Reis I, Meyer PT, Maldjian JA, Gonzalez-Atavales J, et al. Detection of mesial temporal lobe hypoperfusion in patients with temporal lobe epilepsy by use of arterial spin labeled perfusion MR imaging. AJNR Am J Neuroradiol. 2001; 22:1334–1341.
24. Storti SF, Boscolo Galazzo I, Del Felice A, Pizzini FB, Arcaro C, Formaggio E, et al. Combining ESI, ASL and PET for quantitative assessment of drug-resistant focal epilepsy. Neuroimage. 2014; 102(Pt 1):49–59.


25. Boscolo Galazzo I, Storti SF, Del Felice A, Pizzini FB, Arcaro C, Formaggio E, et al. Patient-specific detection of cerebral blood flow alterations as assessed by arterial spin labeling in drug-resistant epileptic patients. PLoS One. 2015; 10:e0123975.


26. Lim YM, Cho YW, Shamim S, Solomon J, Birn R, Luh WM, et al. Usefulness of pulsed arterial spin labeling MR imaging in mesial temporal lobe epilepsy. Epilepsy Res. 2008; 82:183–189.


27. Cianfoni A, Caulo M, Cerase A, Della Marca G, Falcone C, Di Lella GM, et al. Seizure-induced brain lesions: a wide spectrum of variably reversible MRI abnormalities. Eur J Radiol. 2013; 82:1964–1972.


28. Provenzale JM, Barboriak DP, VanLandingham K, MacFall J, Delong D, Lewis DV. Hippocampal MRI signal hyperintensity after febrile status epilepticus is predictive of subsequent mesial temporal sclerosis. AJR Am J Roentgenol. 2008; 190:976–983.


29. Vattipally VR, Bronen RA. MR imaging of epilepsy: strategies for successful interpretation. Magn Reson Imaging Clin N Am. 2006; 14:225–247.


30. Bast T, Ramantani G, Seitz A, Rating D. Focal cortical dysplasia: prevalence, clinical presentation and epilepsy in children and adults. Acta Neurol Scand. 2006; 113:72–81.


31. Stanescu L, Ishak GE, Khanna PC, Biyyam DR, Shaw DW, Parisi MT. FDG PET of the brain in pediatric patients: imaging spectrum with MR imaging correlation. Radiographics. 2013; 33:1279–1303.


32. Wintermark P, Lechpammer M, Warfield SK, Kosaras B, Takeoka M, Poduri A, et al. Perfusion imaging of focal cortical dysplasia using arterial spin labeling: correlation with histopathological vascular density. J Child Neurol. 2013; 28:1474–1482.
33. Wissmeyer M, Altrichter S, Pereira VM, Viallon M, Federspiel A, Seeck M, et al. Arterial spin-labeling MRI perfusion in tuberous sclerosis: correlation with PET. J Neuroradiol. 2010; 37:127–130.


34. Rintahaka PJ, Chugani HT, Messa C, Phelps ME. Hemimegalencephaly: evaluation with positron emission tomography. Pediatr Neurol. 1993; 9:21–28.


35. Altrichter S, Pendse N, Wissmeyer M, Jägersberg M, Federspiel A, Viallon M, et al. Arterial spin-labeling demonstrates ictal cortical hyperperfusion in epilepsy secondary to hemimegalencephaly. J Neuroradiol. 2009; 36:303–305.


36. Wintermark P, Roulet-Perez E, Maeder-Ingvar M, Moessinger AC, Gudinchet F, Meuli R. Perfusion abnormalities in hemimegalencephaly. Neuropediatrics. 2009; 40:92–96.


37. Van Bogaert P, David P, Gillain CA, Wikler D, Damhaut P, Scalais E, et al. Perisylvian dysgenesis. Clinical, EEG, MRI and glucose metabolism features in 10 patients. Brain. 1998; 121(Pt 12):2229–2238.


38. De Volder AG, Gadisseux JF, Michel CJ, Maloteaux JM, Bol AC, Grandin CB, et al. Brain glucose utilization in band heterotopia: synaptic activity of “double cortex”. Pediatr Neurol. 1994; 11:290–294.


39. Seniaray N, Jain A. PET MRI coregistration in intractable epilepsy and gray matter heterotopia. Clin Nucl Med. 2017; 42:e171–e172.


40. Conrad GR, Sinha P. FDG PET imaging of subependymal gray matter heterotopia. Clin Nucl Med. 2005; 30:35–36.


41. Thom M, Blümcke I, Aronica E. Long-term epilepsy-associated tumors. Brain Pathol. 2012; 22:350–379.


42. Radhakrishnan A, Abraham M, Vilanilam G, Menon R, Menon D, Kumar H, et al. Surgery for “long-term epilepsy associated tumors (LEATs)”: seizure outcome and its predictors. Clin Neurol Neurosurg. 2016; 141:98–105.


43. Yeom KW, Mitchell LA, Lober RM, Barnes PD, Vogel H, Fisher PG, et al. Arterial spin-labeled perfusion of pediatric brain tumors. AJNR Am J Neuroradiol. 2014; 35:395–401.


44. Hamer HM, Hong SB. Is an epilepsy presurgical evaluation necessary for mid-grade and high-grade brain tumors presenting with seizures? Epilepsia. 2013; 54:Suppl 9. 56–60.


45. Josephson CB, Rosenow F, Al-Shahi Salman R. Intracranial vascular malformations and epilepsy. Semin Neurol. 2015; 35:223–234.


46. Le TT, Fischbein NJ, André JB, Wijman C, Rosenberg J, Zaharchuk G. Identification of venous signal on arterial spin labeling improves diagnosis of dural arteriovenous fistulas and small arteriovenous malformations. AJNR Am J Neuroradiol. 2012; 33:61–68.


47. Wolf RL, Wang J, Detre JA, Zager EL, Hurst RW. Arteriovenous shunt visualization in arteriovenous malformations with arterial spin-labeling MR imaging. AJNR Am J Neuroradiol. 2008; 29:681–687.


48. Blauwblomme T, Naggara O, Brunelle F, Grévent D, Puget S, Di Rocco F, et al. Arterial spin labeling magnetic resonance imaging: toward noninvasive diagnosis and follow-up of pediatric brain arteriovenous malformations. J Neurosurg Pediatr. 2015; 15:451–458.


49. Yoo RE, Yun TJ, Yoon BW, Lee SK, Lee ST, Kang KM, et al. Identification of cerebral perfusion using arterial spin labeling in patients with seizures in acute settings. PLoS One. 2017; 12:e0173538.


50. Dussaule C, Masnou P, Nasser G, Archambaud F, Cauquil-Michon C, Gagnepain JP, et al. Can developmental venous anomalies cause seizures? J Neurol. 2017; 264:2495–2505.


51. Iv M, Fischbein NJ, Zaharchuk G. Association of developmental venous anomalies with perfusion abnormalities on arterial spin labeling and bolus perfusion-weighted imaging. J Neuroimaging. 2015; 25:243–250.


52. Pinto A, Sahin M, Pearl PL. Epileptogenesis in neurocutaneous disorders with focus in Sturge Weber syndrome. F1000Res. 2016; 5:pii: F1000 Faculty Rev-370.


53. Varadkar S, Cross JH. Rasmussen syndrome and other inflammatory epilepsies. Semin Neurol. 2015; 35:259–268.


54. Hauf M, Wiest R, Nirkko A, Strozzi S, Federspiel A. Dissociation of epileptic and inflammatory activity in Rasmussen encephalitis. Epilepsy Res. 2009; 83:265–268.


55. Kumar S, Nagesh CP, Thomas B, Radhakrishnan A, Menon RN, Kesavadas C. Arterial spin labeling hyperperfusion in Rasmussen's encephalitis: is it due to focal brain inflammation or a postictal phenomenon? J Neuroradiol. 2018; 45:6–14.


56. Graus F, Titulaer MJ, Balu R, Benseler S, Bien CG, Cellucci T, et al. A clinical approach to diagnosis of autoimmune encephalitis. Lancet Neurol. 2016; 15:391–404.


57. Kelley BP, Patel SC, Marin HL, Corrigan JJ, Mitsias PD, Griffith B. Autoimmune encephalitis: pathophysiology and imaging review of an overlooked diagnosis. AJNR Am J Neuroradiol. 2017; 38:1070–1078.


58. Sachs JR, Zapadka ME, Popli GS, Burdette JH. Arterial spin labeling perfusion imaging demonstrates cerebral hyperperfusion in anti-NMDAR encephalitis. Radiol Case Rep. 2017; 12:833–837.


59. Espinosa-Jovel C, Toledano R, García-Morales I, Álvarez-Linera J, Gil-Nagel A. Serial arterial spin labeling MRI in autonomic status epilepticus due to anti-LGI1 encephalitis. Neurology. 2016; 87:443–444.


61. Noguchi T, Yakushiji Y, Nishihara M, Togao O, Yamashita K, Kikuchi K, et al. Arterial spin-labeling in central nervous system infection. Magn Reson Med Sci. 2016; 15:386–394.


62. Ances BM, Sisti D, Vaida F, Liang CL, Leontiev O, Perthen JE, et al. HNRC group. Resting cerebral blood flow: a potential biomarker of the effects of HIV in the brain. Neurology. 2009; 73:702–770.


63. Khoury MN, Gheuens S, Ngo L, Wang X, Alsop DC, Koralnik IJ. Hyperperfusion in progressive multifocal leukoencephalopathy is associated with disease progression and absence of immune reconstitution inflammatory syndrome. Brain. 2013; 136(Pt 11):3441–3450.


64. Topolnik L, Steriade M, Timofeev I. Partial cortical deafferentation promotes development of paroxysmal activity. Cereb Cortex. 2003; 13:883–893.


65. Deibler AR, Pollock JM, Kraft RA, Tan H, Burdette JH, Maldjian JA. Arterial spin-labeling in routine clinical practice, part 2: hypoperfusion patterns. AJNR Am J Neuroradiol. 2008; 29:1235–1241.


66. Miyaji Y, Kawabata Y, Joki H, Seki S, Mori K, Kamide T, et al. Arterial spin-labeling magnetic resonance imaging for diagnosis of early seizure after stroke. J Neurol Sci. 2015; 354:127–128.


67. Miyaji Y, Yokoyama M, Kawabata Y, Joki H, Kushi Y, Yokoi Y, et al. Arterial spin-labeling magnetic resonance imaging for diagnosis of late seizure after stroke. J Neurol Sci. 2014; 339:87–90.


68. Nagesh C, Asranna A, K P D, Cherian A, Nanda S, Thomas B. Culpable brain lesion causing complex partial status in Wilson's disease: deduction by arterial spin labeled perfusion MRI. Seizure. 2017; 46:50–52.


69. So EL, Ryvlin P. MRI-negative epilepsy. 1st ed. Cambridge: Cambridge University Press;2015. p. 255.
70. Wang ZI, Alexopoulos AV, Jones SE, Jaisani Z, Najm IM, Prayson RA. The pathology of magnetic-resonance-imaging-negative epilepsy. Mod Pathol. 2013; 26:1051–1058.


71. Jeon TY, Kim JH, Lee J, Yoo SY, Hwang SM, Lee M. Value of repeat brain MRI in children with focal epilepsy and negative findings on initial MRI. Korean J Radiol. 2017; 18:729–738.


72. Rheims S, Jung J, Ryvlin P. Combination of PET and magnetoencephalography in the presurgical assessment of MRI-negative epilepsy. Front Neurol. 2013; 4:188.


73. Boscolo Galazzo I, Mattoli MV, Pizzini FB, De Vita E, Barnes A, Duncan JS, et al. Cerebral metabolism and perfusion in MR-negative individuals with refractory focal epilepsy assessed by simultaneous acquisition of (18)F-FDG PET and arterial spin labeling. Neuroimage Clin. 2016; 11:648–657.