Abstract
Purpose
Asthma is a chronic inflammatory airway disease characterized by airway hyperresponsiveness (AHR), inflammation, and remodeling. There is emerging interest in the involvement of the transient receptor potential vanilloid 1 (TRPV1) channel in the pathophysiology of asthma. This study examined whether TRPV1 antagonism alleviates asthma features in a murine model of chronic asthma.
Methods
BALB/c mice were sensitized to and challenged by ovalbumin to develop chronic asthma. Capsazepine (TRPV1 antagonist) or TRPV1 small interfering RNA (siRNA) was administered in the treatment group to evaluate the effect of TPV1 antagonism on AHR, airway inflammation, and remodeling.
Results
The mice displayed increased AHR, airway inflammation, and remodeling. Treatment with capsazepine or TRPV1 siRNA reduced AHR to methacholine and airway inflammation. Type 2 T helper (Th2) cytokines (interleukin [IL]-4, IL-5, and IL-13) were reduced and epithelial cell-derived cytokines (thymic stromal lymphopoietin [TSLP], IL-33, and IL-25), which regulate Th2 cytokine-associated inflammation, were also reduced. Airway remodeling characterized by goblet cell hyperplasia, increased α-smooth muscle action, and collagen deposition was also alleviated by both treatments.
Asthma is a chronic inflammatory airway disease characterized by airway hyperresponsiveness (AHR), inflammation, and remodeling.1 The prevalence of asthma has been increasing over the past few decades, and its economic burden is substantial, especially in uncontrolled asthma.2345 It has become a major public health problem. Understanding the precise pathophysiology of asthma is important to achieve optimal management.
The transient receptor potential vanilloid 1 (TRPV1) channel is a non-selective calcium ion (Ca2+) channel that is expressed in various cell types, including sensory neurons, epithelial cells, and smooth muscle cells.67 It is stimulated by various stimuli, including noxious chemicals, low pH, hot temperature, and endogenous mediators.89 Many studies have sought to reveal the role of the TRPV1 channel in airway diseases, with an emphasis on chronic cough. TRPV1 expression in the airway nerves is increased in chronic cough patients, and TRPV1 antagonists may have potential value as antitussive drugs.101112 Mucus hypersecretion and airway inflammation may also be associated with TRPV1 sensitization.13
The role of TRPV1 in the pathophysiology of asthma has attracted attention. TRPV1 expression is increased in the airway epithelium of asthmatic patients and is more prominent in severe, uncontrolled disease.14 However, the roles of TRPV1 and the effects of TRPV1 antagonism on airway inflammation in animal models are debatable. Rehman et al.15 reported that inhibition of TRPV1 reduced AHR and airway remodeling in interleukin (IL)-13-induced asthma model in BALB/c mice. However, Caceres et al.16 induced an acute asthma murine model in genetically silenced C57BL/6 mice using ovalbumin (OVA) and obtained negative results. The results of TRPV1 blocking are diverse in different experimental materials and study settings.
In addition to the contrary results of TRPV1 antagonism in diverse murine asthma models, there was also lack of studies performed in a chronic asthma model compared to acute model. Chronic asthma model may demonstrate better asthma features, especially airway remodeling, which is important in human asthma pathophysiology.
In this study, we investigated the role of TRPV1 in the airway of a murine model of chronic asthma. We also examined whether TRPV1 suppression by inhalation of antagomir, a small interfering RNA (siRNA) directed toward TPRV1, may alleviate pathologic manifestation of chronic asthma compared to the well-known TRPV1 inhibitor capsazepine.
Six-week-old female BALB/c mice (Orient Bio Inc., Seongnam, Korea) were randomly allocated to the following groups: 1) control, 2) OVA challenge, 3) OVA challenge plus capsazepine, or 4) OVA plus TRPV1 siRNA.
Sensitization and antigen challenge with OVA were performed as previously described.1718 Mice were immunized by subcutaneously injecting 25 µg of OVA (Grade V; Sigma-Aldrich, St. Louis, MO, USA) absorbed to 1 mg of aluminum hydroxide (Aldrich, Milwaukee, WI, USA) in 200 µL of phosphate-buffered saline (PBS). Subcutaneous injections were administered on days 0, 7, 14, and 21, followed by intranasal OVA challenge (20/50 µL in PBS) performed on days 33 and 35. Subsequently, intranasal OVA challenges were repeated twice per week for 3 months. Age- and gender-matched control mice were treated equally with PBS. All procedures were performed while mice were anesthetized using isoflurane (Vedco, St. Joseph, MO, USA). Mice were sacrificed 24 hours after the final intranasal OVA challenge, and bronchoalveolar lavage (BAL) fluid and lung tissues were obtained for analysis.
All animal procedures were performed in accordance with Laboratory Animal Welfare Act, the Guide for the Care and Use of Laboratory Animals, and the Guidelines and Policies for Rodent Experiments provided by Institutional Animal Care and Use Committee at the School of Medicine, The Catholic University of Korea (approval number: CUMC-2015-0194-04).
Capsazepine (Cayman, Ann Arbor, MI, USA) was given 50 µg once daily for 3 months by intraperitoneal injection starting on day 38. TRPV1 siRNA (Bioneer, Daejeon, Korea) was also administrated intranasally 50 µg 2 times per week once per day beginning on day 38 for 3 months, during OVA challenge. The control mice were treated identically with normal saline.
AHR to methacholine (Mch)(Sigma-Aldrich) was assessed 24 hours after the final OVA challenge with the flexiVent system (SCIREQ, Montreal, Canada) as previously described.19 Briefly, mice were anesthetized with an intraperitoneal administration of a 1:4 mixture of rompun and zoletil. The trachea was exposed and cannulated to connect it with a computer-controlled small-animal ventilator. Ventilation was applied with a tidal volume of 10 mL/kg at a frequency of 150 breaths/min and a positive end-expiratory pressure of 2 cm H2O, which was close to the mean lung volume of mouse spontaneous breathing. Each mouse was challenged with PBS control, followed by Mch aerosol with increasing concentrations (6.25, 12.5, 25, and 50 mg/mL). Changes in airway resistance with increasing concentrations of inhaled Mch were measured.
Mice were sacrificed by CO2 asphyxiation after measurement of AHR. The trachea was exposed and cannulated with a silicone tube attached to a 23-gauge needle on a 1-mL tuberculin syringe. BAL was performed by instillation of 0.8 mL of sterile PBS through the trachea into the lung. The total cell counts in BAL fluid were analyzed using a LUNA™ Automated Cell Counter (Logos Biosystems, Inc., Annandale, VA, USA).
The BAL fluid was cytospun at 2,000 rpm for 7 minutes, placed on microscope slides, and stained with Diff-Quick (Sysmax, Kobe, Japan). The percentages of macrophages, eosinophils, lymphocytes, and neutrophils in the BAL fluid were calculated by counting 500 leukocytes on randomly selected areas of the slide using light microscopy. Supernatants were stored at −70℃.
After BAL was performed, the mouse lungs were inflated, fixed in 4% paraformaldehyde for 24 hours, and embedded in paraffin using a standard protocol. Sections were cut 4-µm thick using a microtome and stained with hematoxylin and eosin (H&E). Paraffin-embedded tissues were also sectioned and the 5- to 6-µm thick sections were stained with periodic acid-Schiff (PAS) to distinguish goblet cells in the epithelium. Goblet cell hyperplasia was quantified as previously described.20 The pathological changes were evaluated according to a modified 5-point scoring system (grades 0–4) based on the percentage of goblet cells in the epithelium: grade 0 (no goblet cells), grade 1 (<25%), grade 2 (25%–50%), grade 3 (51%–75%), and grade 4 (>75%). The mean goblet cell hyperplasia score was then calculated for each mouse.
The concentrations of IL-4, IL-5, and IL-13 were measured in BAL fluid. Concentrations of IL-17E (IL-25), IL-33, and thymic stromal lymphopoietin (TSLP) were measured with lung homogenate with an ELISA kit (R&D Systems, Minneapolis, MN, USA). The assay was performed according to the manufacturer's protocol.
Six-µm-thick lung sections from each paraffin block were deparaffinized with xylene and rehydrated in ethanol. For immunohistochemical detection of α-smooth muscle actin (α-SMA) and TRPV1, the lung sections were incubated overnight at 4℃ with a primary monoclonal antibody against α-SMA (titer 1:25; Abcam, Cambridge, MA, USA) and TRPV1 (titer 1:100; Abcam), or mouse serum as a negative control instead of the primary antibody. Immunoreactivity was detected by sequential incubations of lung sections with a biotinylated secondary antibody, followed by peroxidase reagent and the 3,3′-diaminobenzidine (DAB) chromogen (Invitrogen, Carlsbad, CA, USA). The area in each paraffin-embedded lung immunostained by α-SMA was outlined and quantified using a light microscope attached to an image analysis system (BX50; Olympus, Tokyo, Japan). The results were expressed as the immunostained area of the bronchiolar basement membrane (internal diameter 150–200 µm). At least 10 bronchioles were counted in each slide.
Lung tissue (60 mg) from each mouse was used for the hydroxyproline assay with hydroxyproline colorimetric assay kit (BioVision, Milpitas, CA, USA) according to the manufacturer's instructions. Hydroxyproline concentrations were calculated from a standard curve of hydroxyproline.
The results from each group were analyzed by analysis of variance (ANOVA) and the nonparametric Kruskal-Wallis test. All statistical analyses were performed using Graph-Pad Prism for Windows software (ver. 5.00; GraphPad Software, San Diego, CA, USA). A P value of <0.05 was considered statistically significant. All results are expressed as mean±standard error of the mean (SEM).
The OVA challenge group showed increased AHR compared to the control group at Mch doses of 25 and 50 mg/dL. The OVA plus capsazepine group displayed a remarkable decrease in airway resistance at 50 mg/mL Mch. Airway resistance was significantly decreased in mice treated with TRPV1 siRNA at Mch doses of 25 and 50 mg/dL (Fig. 1).
Three months of OVA challenge induced a significant increase in number of total cells, macrophages, and eosinophils in the BAL fluid. Treatment with either capsazepine or TRPV1 siRNA significantly reduced the number of total cells, macrophages, and eosinophils in BAL fluid (Fig. 2). Three months of OVA challenge significantly increased the levels of type 2 T helper (Th2)-associated cytokines (IL-3, IL-5, and IL-13) in BAL fluid (Fig. 3A). Mice treated with capsazepine displayed significant decreases in IL-4, IL-5, and IL-13. Treatment with TRPV1 siRNA significantly decreased IL-4 and IL-5. We evaluated the epithelial cell-derived cytokines IL-17E (IL-25), IL-33, and TSLP in the lung homogenate. The OVA challenge group showed increases in all the 3 cytokines compared to the control group (Fig. 3B). Mice treated with either capsazepine or TRPV1 siRNA showed significantly decreased levels of IL-33 and TSLP. However, IL-17E level was decreased in both group, without statistical significance.
Histopathology of H&E-stained sections revealed increased infiltration of subepithelial, peribronchial, and perivascular inflammatory cells, including eosinophils, in the OVA challenge group compared to the control group. In contrast, treatment with either capsazepine or TRPV1 siRNA reduced the inflammatory cell recruitment (Fig. 4A). Immunostaining of TRPV1 showed that chronic OVA challenge increased the area of TRPV1 immunostaining compared to the control group. This effect was alleviated by capsazepine or TRPV1 (Fig. 4B). PAS-stained sections revealed prominent goblet cell hyperplasia in the OVA challenge group. The hyperplasia was attenuated by both treatments (Fig. 5A). The pathologic score was significantly higher in the OVA group compared to both treatment groups (Fig. 5B). Quantification of peribronchial α-SMA immunostaining was performed by imaging (Fig. 6). Chronic OVA challenge induced an increase in the area of peribronchial α-SMA compared to the control group. Treatment with capsazepine or TRPV1 siRNA reduced the area of peribronchial α-SMA. Pulmonary fibrosis was evaluated by measuring the total lung collagen level by using hydroxyproline analysis. Chronic OVA challenge for 3 months resulted in a significant increase in hydroxyproline content (Fig. 7). Capsazepine treatment significantly decreased the level of hydroxyproline compared to the OVA challenge group. TRPV1 siRNA treatment decreased the hydroxyproline level, without statistical significance.
This study revealed that expression of the TRPV1 receptor was significantly elevated in OVA challenged asthmatic lungs and that inhalation of TRPV1 antagomir effectively suppressed eosinophilic airway inflammation and remodeling. The underlying mechanisms of these effects may be associated with suppression of epithelial-derived cytokines, including TSLP, IL-33, and IL-25. This is the first report that the inhibition of the TRPV1 receptor by TRPV1 antagomir inhalation has significant anti-asthmatic effects that are comparable to the established TRPV1 antagonist, capsazepine.
The 3-month chronic OVA-challenged model is unique in that it results in features that are more similar to human asthma than the acute model, which features airway remodeling, including airway smooth muscle cell (ASMC) proliferation, goblet cell hyperplasia, and collagen deposition. The present results are important since, to our knowledge, no prior study has explored the role of TRPV1 in a chronic allergic asthma animal model.
Asthma is an allergic inflammatory airway disease in which pathogenesis involves various inflammatory cells and mediators. The most important cellular pathway of asthma starts with recognition of allergen by dendritic cells in airway epithelium and submucosa.21 As dendritic cells function as antigen-presenting cells to Th2 lymphocytes, Th2 cytokines are released. This release induces eosinophils and mast cells to initiate allergic inflammation.22 In this process, bronchial epithelial cells play an important role in asthma pathophysiology. They function as a mechanical barrier from allergens and other toxic materials through tight junctions, and also release cytokines—including IL-25, IL-33, TSLP, and granulocyte macrophage-colony stimulating factor—or endogenous danger signals in response to various allergens to modulate allergic inflammation.23
The TRPV1 channel is expressed in both sensory neurons and non-neuronal cells, including bronchial epithelium, smooth muscle cells, mast cells, and dendritic cells.811142425 Consistent with a previous study, we identified increased TRPV1 expression of epithelial cells by immunochemical staining in the murine asthma model. Activation of TRPV1 in bronchial epithelial cells stimulates the release of proinflammatory mediators, including ILs, prostaglandin E2, nerve growth factor, and tumor necrosis factor-α, to promote airway inflammation and airway hypersensitivity.62627
Recent studies have demonstrated that TRPV1 is important in the pathway of airway inflammation and remodeling, which is a key pathologic feature of asthma.28293031 Earlier studies reported that inhibition of TRPV1 reduced airway constriction using capsazepine and in a knockout animal model. Ellis and Undem32 revealed that capsazepine inhibits capsaicin-sensitive receptors that attenuate airway constriction. Furthermore, Watanabe et al.33 demonstrated that loss of TRPV1-positive axon results in alleviation of bronchoconstriction. Also, there are some studies that explored the role of TRPV1 receptor in asthma animal models. The findings have been inconsistent. Rehman et al.15 observed that TRPV1 siRNA treatment in acute asthma murine model significantly alleviates asthma features. Also, Baker et al.25 reveal attenuation of asthma features in genetically silenced TRPV1, although the results did not reach statistical significance. Caceres et al.16 reported that TRPV1 knockout mice showed no difference, compared to the wild type, in AHR or airway inflammation in an OVA-challenged asthma model. Raemdonck et al.34 demonstrated a similar result that asthma was not attenuated by the TRPV1 blockers JNJ-17203212 and capsazepine in an OVA-induced murine asthma model. Moreover, Mori et al.35 suggested that TRPV1 gene knockout mice intensified AHR and airway inflammation compared to the wild type in an asthma model, which is the exactly the opposite result of our study. These conflicting results may reflect the mouse strains used; the prior study involved C57BL/6 mice. Distribution of capsaicin receptors varies with strain, and there may be less TRPV1 expression in C57BL/6 mice than in BALB/c mice.1536
Currently, TRPV1 expression was significantly elevated in lung tissues of the OVA-induced asthma model. In addition, we confirmed that capsazepine (TRPV1 antagonist) and TRPV1 siRNA (antagomir) inhalation resulted in the attenuation of AHR and inflammation. Antagomir inhalation for 3 months showed noninferior effects compared to capsazepine.
In this study, we found that anti-inflammatory effects on the asthmatic airway may be associated with decreased expression of epithelial-derived cytokines and Th2 cytokines by TRPV1 blocking. Epithelial cell-derived cytokines, such as TSLP, IL-33, and IL-25, are important regulators of Th2 cytokine associated inflammation.3738 IL-33 and IL-25 activate Th2 lymphocytes and other cells, which mediate allergic reactions and activate TSLP production. TSLP is important in the activation of dendritic cells and naïve T cells. In our study, the levels of IL-33 and TSLP were significantly decreased after TRPV1 blocking. Also, IL-25 displayed a decreasing trend, which did not reach statistical significance. These results imply that TRPV1 channel may play a crucial role in bronchial epithelial cells after chronic OVA challenge and affect Th2-mediated inflammation by regulating epithelial cell-derived cytokine expression. However, the limitation of our study is that exact mechanism underlying down-regulation of IL-33 and IL-25 by TRPV1 blocking have not been revealed. Further studies are needed to reveal the role of TRPV1 on airway epithelial cells and other inflammatory cells, including in vitro experiment using the epithelial cell line.
Airway remodeling is an essential pathophysiologic feature of chronic bronchial asthma.39 Currently, TRPV1 antagonism alleviated airway remodeling by reducing airway smooth muscle thickening and collagen deposition. Until now, the effect of TRPV1 inhibition on airway remodeling has not been fully understood. One of the most important features of airway remodeling is ASMC hypertrophy and hyperplasia.22 Zhao et al.28 revealed that the TRPV1 channel is overexpressed and activated in ASMCs of asthmatic rats. Treatment with capsaicin has been reported to enhance ASMC proliferation and decrease apoptosis, whereas capsazepine did in an opposite manner. ASMC hypertrophy and hyperplasia are important features of airway remodeling. Thus, the TRPV1 channel in ASMCs may play a crucial role in airway remodeling in asthma. Furthermore, the accumulation of matrix proteins, such as collagen fibers, is also responsible for airway thickening in chronic asthma.22 A previous study revealed that, although TRPV1 channel is not generally expressed in airway fibroblasts, it is expressed significantly in inflammatory conditions induced by tumor necrosis factor-α, lipopolysaccharide, and IL-1α.26 This result suggests that bronchial fibroblasts may be activated to synthesize collagen fibers in inflammatory conditions, such as bronchial asthma, via the TRPV1 channel. Further studies are needed to understand the exact mechanism of TRPV1 in airway remodeling.
In conclusion, blocking the TRPV1 pathway by capsazepine or TRPV1 siRNA inhalation attenuates OVA-mediated asthma features, including allergic inflammation, AHR, and airway remodeling. The TRPV1 antagonist delivered via nasal inhalation might have therapeutic potential in the treatment of bronchial asthma.
Figures and Tables
Fig. 1
Effect of capsazepine and TRPV1 siRNA on AHR to Mch. AHR was measured 24 hours after the final OVA challenge with the flexiVent system. Mch concentration was increased from 6.25 to 100 mg/mL. The values are expressed as mean±SEM (n=4–8/group). TRPV1, transient receptor potential vanilloid 1; siRNA, small interfering RNA; AHR, airway hyperresponsiveness; Mch, methacholine; OVA, ovalbumin; SEM, standard error of the mean. *P<0.01, †P<0.001 compared to control, ‡P<0.01, §P<0.001 compared to the OVA group.
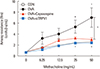
Fig. 2
Effect of capsazepine and TRPV1 siRNA on total/differential cell counts in BAL fluid. Mice were sacrificed after measurement of AHR, and BAL was analyzed immediately. The values are expressed as mean±SEM (n=4–8/group). TRPV1, transient receptor potential vanilloid 1; siRNA, small interfering RNA; BAL, bronchoalveolar lavage; AHR, airway hyperresponsiveness; SEM, standard error of the mean; OVA, ovalbumin. *P<0.001 compared to control, †P<0.001 compared to the OVA group.
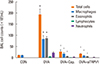
Fig. 3
Effect of capsazepine and TRPV1 siRNA on levels of Th2 cytokines and epithelial-derived cytokines. (A) The concentration of IL-4, IL-5, and IL-13 were measured in the BAL fluid with and ELISA. (B) Levels of IL-17E, IL-33, and TSLP in the lung homogenates were measured. The values are expressed as mean±SEM (n=4–8/group). TRPV1, transient receptor potential vanilloid 1; siRNA, small interfering RNA; Th2, type 2 T helper; IL, interleukin; BAL, bronchoalveolar lavage; ELISA, enzyme-linked immunosorbent assay; TSLP, thymic stromal lymphopoietin; SEM, standard error of the mean; OVA, ovalbumin. *P<0.01, †P<0.001 compared to control, ‡P<0.05, §P<0.01, ∥P<0.001 compared to the OVA group for (A). ¶P<0.05, **P<0.001 compared to control, ††P<0.05, ‡‡P<0.001 compared to the OVA group for (B).
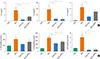
Fig. 4
Effect of capsazepine and TRPV1 siRNA on perbronchial inflammation and TRPV1 receptor expression in lung tissues. (A) Lung tissues were fixed with 4% paraformaldehyde and embedded in paraffin. Paraffin block were sectioned (4-µm thick) and stained with H&E (×200). (B) The TRPV1 receptor was immunostained in paraffin-embedded lung section (blue). TRPV1, transient receptor potential vanilloid 1; siRNA, small interfering RNA; H&E, hematoxylin and eosin; Br, bronchus; Bm, basement membrane; Eo, eosinophil; Ep, epithelium; Bl, blood vessel.
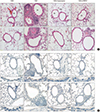
Fig. 5
Effect of capsazepine and TRPV1 siRNA on goblet cell hyperplasia in lung tissues. (A) The paraffin-embedded tissues were cut into 5- to 6-µm-thick sections and stained with PAS. (B) Goblet cell hyperplasia was quantified using a modified 5-point scoring system, based on the percentage of goblet cells in the epithelium: grade 0 (no goblet cells), grade 1 (<25%), grade 2 (25%–50%), grade 3 (51%–75%), and grade 4 (>75%). The values are expressed as mean±SEM (n=4–8/groups). TRPV1, transient receptor potential vanilloid 1; siRNA, small interfering RNA; PAS, periodic acid-Schiff; OVA, ovalbumin. *P<0.001 compared to control, †P<0.001 compared to the OVA group.
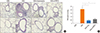
Fig. 6
Effect of capsazepine and TRPV1 siRNA on the area of peribronchial airway smooth muscle. (A) Peribronchial α-SMA was immunostained in paraffine-embedded lung section. (B) The immunostained area was quantified by using a light microscope attached to BX50. The values are expressed as mean±SEM (n=4–8/group). TRPV1, transient receptor potential vanilloid 1; siRNA, small interfering RNA; α-SMA, α-smooth muscle actin; SEM, standard error of the mean; OVA, ovalbumin. *P<0.01 compared to control, †P<0.01 compared to the OVA group.
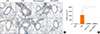
Fig. 7
Effect of capsazepine and TRPV1 siRNA on total collagen levels. Lung tissue was collected from each mouse for the hydroxyproline assay. The values are expressed as mean±SEM (n=4–8/groups). TRPV1, transient receptor potential vanilloid 1; siRNA, small interfering RNA; SEM, standard error of the mean; OVA, ovalbumin; HYP, hydroxyproline. *P<0.01 compared to control, †P<0.01 compared to the OVA group.
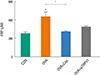
References
1. Rosano A, Loha CA, Falvo R, van der Zee J, Ricciardi W, Guasticchi G, et al. The relationship between avoidable hospitalization and accessibility to primary care: a systematic review. Eur J Public Health. 2013; 23:356–360.


2. Bedouch P, Sadatsafavi M, Marra CA, FitzGerald JM, Lynd LD. Trends in asthma-related direct medical costs from 2002 to 2007 in British Columbia, Canada: a population based-cohort study. PLoS One. 2012; 7:e50949.


3. Akinbami LJ, Moorman JE, Bailey C, Zahran HS, King M, Johnson CA, et al. Trends in asthma prevalence, health care use, and mortality in the United States, 2001–2010. NCHS Data Brief. 2012. p. 1–8.
4. Fajt ML, Wenzel SE. Development of new therapies for severe asthma. Allergy Asthma Immunol Res. 2017; 9:3–14.


5. Yoo KH, Ahn HR, Park JK, Kim JW, Nam GH, Hong SK, et al. Burden of respiratory disease in Korea: an observational study on allergic rhinitis, asthma, COPD, and rhinosinusitis. Allergy Asthma Immunol Res. 2016; 8:527–534.


6. Zholos AV. TRP channels in respiratory pathophysiology: the role of oxidative, chemical irritant and temperature stimuli. Curr Neuropharmacol. 2015; 13:279–291.


7. Adcock JJ. TRPV1 receptors in sensitisation of cough and pain reflexes. Pulm Pharmacol Ther. 2009; 22:65–70.


8. Grace MS, Baxter M, Dubuis E, Birrell MA, Belvisi MG. Transient receptor potential (TRP) channels in the airway: role in airway disease. Br J Pharmacol. 2014; 171:2593–2607.


10. Groneberg DA, Niimi A, Dinh QT, Cosio B, Hew M, Fischer A, et al. Increased expression of transient receptor potential vanilloid-1 in airway nerves of chronic cough. Am J Respir Crit Care Med. 2004; 170:1276–1280.


11. Mitchell JE, Campbell AP, New NE, Sadofsky LR, Kastelik JA, Mulrennan SA, et al. Expression and characterization of the intracellular vanilloid receptor (TRPV1) in bronchi from patients with chronic cough. Exp Lung Res. 2005; 31:295–306.


12. McLeod RL, Correll CC, Jia Y, Anthes JC. TRPV1 antagonists as potential antitussive agents. Lung. 2008; 186:Suppl 1. S59–S65.


13. Yang J, Yu HM, Zhou XD, Kolosov VP, Perelman JM. Study on TRPV1-mediated mechanism for the hypersecretion of mucus in respiratory inflammation. Mol Immunol. 2013; 53:161–171.


14. McGarvey LP, Butler CA, Stokesberry S, Polley L, McQuaid S, Abdullah H, et al. Increased expression of bronchial epithelial transient receptor potential vanilloid 1 channels in patients with severe asthma. J Allergy Clin Immunol. 2014; 133:704–712.e4.


15. Rehman R, Bhat YA, Panda L, Mabalirajan U. TRPV1 inhibition attenuates IL-13 mediated asthma features in mice by reducing airway epithelial injury. Int Immunopharmacol. 2013; 15:597–605.


16. Caceres AI, Brackmann M, Elia MD, Bessac BF, del Camino D, D'Amours M, et al. A sensory neuronal ion channel essential for airway inflammation and hyperreactivity in asthma. Proc Natl Acad Sci U S A. 2009; 106:9099–9104.


17. Coyle AJ, Lloyd C, Tian J, Nguyen T, Erikkson C, Wang L, et al. Crucial role of the interleukin 1 receptor family member T1/ST2 in T helper cell type 2-mediated lung mucosal immune responses. J Exp Med. 1999; 190:895–902.


18. Lee HY, Kim IK, Yoon HK, Kwon SS, Rhee CK, Lee SY. Inhibitory effects of resveratrol on airway remodeling by transforming growth factor-β/Smad signaling pathway in chronic asthma model. Allergy Asthma Immunol Res. 2017; 9:25–34.


19. Tarkowski M, Vanoirbeek JA, Vanhooren HM, De Vooght V, Mercier CM, Ceuppens J, et al. Immunological determinants of ventilatory changes induced in mice by dermal sensitization and respiratory challenge with toluene diisocyanate. Am J Physiol Lung Cell Mol Physiol. 2007; 292:L207–L214.


20. Padrid P, Snook S, Finucane T, Shiue P, Cozzi P, Solway J, et al. Persistent airway hyperresponsiveness and histologic alterations after chronic antigen challenge in cats. Am J Respir Crit Care Med. 1995; 151:184–193.


21. von Garnier C, Filgueira L, Wikstrom M, Smith M, Thomas JA, Strickland DH, et al. Anatomical location determines the distribution and function of dendritic cells and other APCs in the respiratory tract. J Immunol. 2005; 175:1609–1618.


23. Lambrecht BN, Hammad H. Allergens and the airway epithelium response: gateway to allergic sensitization. J Allergy Clin Immunol. 2014; 134:499–507.


24. Caterina MJ, Schumacher MA, Tominaga M, Rosen TA, Levine JD, Julius D. The capsaicin receptor: a heat-activated ion channel in the pain pathway. Nature. 1997; 389:816–824.


25. Baker K, Raemdonck K, Dekkak B, Snelgrove RJ, Ford J, Shala F, et al. Role of the ion channel, transient receptor potential cation channel subfamily V member 1 (TRPV1), in allergic asthma. Respir Res. 2016; 17:67.


26. Sadofsky LR, Ramachandran R, Crow C, Cowen M, Compton SJ, Morice AH. Inflammatory stimuli up-regulate transient receptor potential vanilloid-1 expression in human bronchial fibroblasts. Exp Lung Res. 2012; 38:75–81.


27. Yu H, Li Q, Zhou X, Kolosov VP, Perelman JM. Transient receptor potential vanilloid 1 receptors mediate acid-induced mucin secretion via Ca2+ influx in human airway epithelial cells. J Biochem Mol Toxicol. 2012; 26:179–186.


28. Zhao L, Zhang X, Kuang H, Wu J, Guo Y, Ma L. Effect of TRPV1 channel on the proliferation and apoptosis in asthmatic rat airway smooth muscle cells. Exp Lung Res. 2013; 39:283–294.


29. Zhao LM, Kuang HY, Zhang LX, Wu JZ, Chen XL, Zhang XY, et al. Effect of TRPV1 channel on proliferation and apoptosis of airway smooth muscle cells of rats. J Huazhong Univ Sci Technolog Med Sci. 2014; 34:504–509.


30. Liu H, Fan X, Wang N, Zhang Y, Yu J. Exacerbating effects of PM2.5 in OVA-sensitized and challenged mice and the expression of TRPA1 and TRPV1 proteins in lungs. J Asthma. 2017; 54:807–817.


31. Helyes Z, Elekes K, Németh J, Pozsgai G, Sándor K, Kereskai L, et al. Role of transient receptor potential vanilloid 1 receptors in endotoxin-induced airway inflammation in the mouse. Am J Physiol Lung Cell Mol Physiol. 2007; 292:L1173–L1181.


32. Ellis JL, Undem BJ. Inhibition by capsazepine of resiniferatoxin- and capsaicin-induced contractions of guinea pig trachea. J Pharmacol Exp Ther. 1994; 268:85–89.
33. Watanabe N, Horie S, Michael GJ, Keir S, Spina D, Page CP, et al. Immunohistochemical co-localization of transient receptor potential vanilloid (TRPV)1 and sensory neuropeptides in the guinea-pig respiratory system. Neuroscience. 2006; 141:1533–1543.


34. Raemdonck K, de Alba J, Birrell MA, Grace M, Maher SA, Irvin CG, et al. A role for sensory nerves in the late asthmatic response. Thorax. 2012; 67:19–25.


35. Mori T, Saito K, Ohki Y, Arakawa H, Tominaga M, Tokuyama K. Lack of transient receptor potential vanilloid-1 enhances Th2-biased immune response of the airways in mice receiving intranasal, but not intraperitoneal, sensitization. Int Arch Allergy Immunol. 2011; 156:305–312.


36. Veronesi B, Oortgiesen M, Roy J, Carter JD, Simon SA, Gavett SH. Vanilloid (capsaicin) receptors influence inflammatory sensitivity in response to particulate matter. Toxicol Appl Pharmacol. 2000; 169:66–76.


37. Saenz SA, Taylor BC, Artis D. Welcome to the neighborhood: epithelial cell-derived cytokines license innate and adaptive immune responses at mucosal sites. Immunol Rev. 2008; 226:172–190.

