Abstract
In the developed and developing world, opioid consumption in combination with alcohol has become one of the substances abused. In this experiment, we examined the effects of alcohol, morphine, and morphine+alcohol combination on cognitive functions and neuroinflammatory responses in the medial prefrontal cortex (mPFC) of juvenile male rats. Alcohol (1.0 ml of 15% v/v ethanol twice daily, subcutaneously, 7 hours apart), morphine (0.5 ml/kg of 0.4 mg/kg morphine chlorate twice daily, subcutaneously, 7 hours apart), morphine+alcohol co-treatment (0.5 ml/kg of 0.4 mg/kg morphine chlorate+1.0 ml of 15% v/v ethanol twice daily, subcutaneously, 7 hours apart) were administered for 21 days. Treatment with morphine+alcohol significantly impairs cognition functions in the Morris water maze, passive avoidance, and novel object recognition tests, furthermore, the treatment significantly increased the quantitative count of astrocytic cells and also conferred marked neuronal cell death in the mPFC, which were studied by glial fibrillary acidic protein immunochemistry for astrocytes and Cresyl violet for Nissl's substance distribution in neurons respectively. These results suggest that alcohol, morphine, and morphine+alcohol co-treatment may trigger cognitive deficits and neuroinflammatory responses in the brain.
The misuse and adverse effects of illicit opioids and diverted pharmaceutical opioids are on the increase in the developed and developing worlds. Addiction to substance abuse is a significant “socio-econo-medical” menace in the developed and developing worlds. It often leads to increase in crime rate, negative psychological impact on the family, aggravates physical discomfort, increases direct medical cost, exacerbates premature mortality and disability [1]. Addiction and misuse of substances abuse impair the functional integrity of all the systems in the body. The major collection of drugs most likely to produce addiction are alcohol, marijuana, nicotine, opiates, phencyclidine-like and psychostimulant drugs.
Morphine is an important opioid ligand used as a pain reliever because of its ability to activate opioid receptor both in the central nervous system and peripheral nervous system [2]. The pharmacological actions of morphine are basically mediated through specialized opioid receptors (µ, δ, and κ receptors) situated in different regions and types of brain cells like neuronal and glial cells [23]. Martini and Whistler (2007) [4], are of the opinion that morphine is capable of mediating several effects in the central nervous system (CNS), leading to analgesia and other effects such as drug addiction, tolerance, and dependence. However, its underlying effects remains controversial.
Over the years, there has been a significant advancement in the pattern of alcohol consumption among teenagers [5]. A substantial body of evidence in human and experimental animals has demonstrated the vulnerability of the central nervous system to the effects of ethanol and that exposure to ethanol during brain ontogenetic development can cause irreversible morphological and functional aberrations on several brain structures and regions [67]. Studies using magnetic resonance imaging have clearly shown that the mammalian brain continues to develop throughout adolescence and into adulthood and that the brain undergoes important structural and functional changes in synaptic plasticity and neural connectivity during the juvenile and adolescence periods [8910].
Most of the neurotoxicity deviations associated with adolescent or juvenile exposure to substance abused occur simultaneously with alterations in the functional integrity of neurotransmitter systems, which significantly determine the excellent functions of certain brain areas and neural circuits [1112]. For instance, the deleterious effects of ethanol have been substantiated in many animal studies, thus providing further evidence of the vulnerability of the juvenile brain to the deleterious effects of ethanol and the long-term cognitive consequences [131415].
The prefrontal cortex (PFC) is hypothetically described as the region of the cortex that receives thalamic inputs from the mediodorsal nucleus of the thalamus. It is situated “somewhere” at the anterior end of the cerebral hemispheres and refers not to a single region but to a group of related regions [16]. The medial prefrontal cortex (mPFC) is one of the regions of the PFC. It is speculated to be associated with functions such as decision making and conflict monitoring, error detection, executive control, reward-guided learning, as well as decision making about risk and reward [171819202122]. The cells in the mPFC are implicated in motoric and sensory events [23].
In many of the developing and developed world, young individuals are prone to consuming alcohol and in combination with some other substances such as opiates, cannabis, hallucinogens, cocaine- and amphetamine-type stimulants, and other various club or date drugs [24]; however, there is insufficient data on the effects of morphine and its possible association with alcohol on the brain and its associated topographies, hence this study. The objective of the study was to evaluate the cognitive and neuropathological changes in the mPFC of juvenile male rats exposed to a morphine-alcohol combination.
All the experimental protocols reported in this study were carried out according to the approved ethical guidelines for the scientific use of animals in research outlined by the Health Research Ethics Committee (HREC), College of Health Sciences, Osun State University (Osogbo, Nigeria) which parallels with the approved National Guidelines for Animal Care. Efforts were made to reduce animal pain and suffering and the number of rats used in this study.
A total of 40 male Wistar rats (postnatal day [PND] 28) were used for the experiment which spans a period of 21 days. The rats were breed in the breeding unit of the animal house in the College of Health Sciences, Osun State University, Osogbo, Nigeria. The rats were fed standard rat-pellet and were given drinking water ad-libitum. The temperature of the room was maintained at 24±1℃ with natural light cycle and humidity of 53±12%. The rats were weighed and randomly assigned into one of the following groups; vehicle, alcohol-treated, morphine-treated, and morphine-alcohol.
The vehicle group (n=10 rats) were subcutaneously administered with double distilled water twice daily (7 hours apart); alcohol-treated group (n=10) were subcutaneously administered with 1.0 ml of 15% v/v ethanol twice daily (7 hours apart); morphine-treated group (n=10) were subcutaneously administered with 0.5 ml/kg of 0.4 mg/kg morphine chlorate twice daily (7 hours apart), and the morphine+alcohol–treated group (n=10) were subcutaneously administered with a combination of 0.5 ml/kg of 0.4 mg/kg morphine chlorate+1.0 ml of 15% v/v ethanol twice daily (7 hours apart). The duration of treatments was 21 days. Twenty-four hours after the last administration of the last respective doses (PND 50), five rats from each group were euthanized and perfused for cytochemical or immunocytochemical studies while the remaining five rats were exposed to the behavioural tests (Fig. 1).
Twenty-four hours after the last injection, 20 rats (five rats from each group) were exposed to the following behavioural tests: Morris water maze, passive avoidance, and novel object recognition. All behavioural trials were recorded with Noldus Ethovision XT (Noldus BV, Waacheningen, Holland).
Morris water maze test was used to evaluated spatial learning and memory function in the rats in the various treatment groups. The method used was according to the method (modified) published by Park et al. [25]. Briefly, an improvised plastic circular basin (90.0 cm in diameter and 45.0 cm height) filled with milky-water was partitioned into four equal quadrants, and a platform (6.0 cm in diameter and 29.0 cm in height) was placed in one quadrant 1.0 cm below the water surface. Four learning trial-trainings was conducted on PND 50, and the probe-test was conducted on PND 51. The rats were allowed to swim for 120 seconds to search for the hidden platform. If they failed to locate the platform within 120 seconds, escape would be assisted, and escape latency was recorded as 120 seconds. At the end of each trial, each rat would stay on the platform for 3 seconds. After the training, the time required for individual rats to find the submerged platform within 120 seconds (escape latency).
Passive avoidance test was used to measure short-term memory in the treated rats from the different groups in this study. The test was performed according to the modified procedure published by Lee et al. [26] and Kim and Park [27]. Briefly, the procedure utilized an apparatus that consisted of light and dark compartments lined with white Formica. The test was divided into a training session (PND 52) and a test session (PND 53). Before introducing the next rat into the apparatus, the chambers were wiped clean with 5% ethanol and dried with a clean handkerchief. In the training session, the door between the compartments was kept open, and rats were allowed to explore the dark compartments for 120 seconds. Then lights were turned on in one compartment, and rats were allowed to explore environments in both light and dark compartments for 120 seconds. Subsequently, the rats were given an inescapable foot shock (0.3 mA for 3 seconds) after entering the dark compartment by closing the door between the two compartments. The test session was done 15 minutes after the training session using the same method employed during the training session but without the foot shock. The time interval between the start of the test session and their entry into the dark compartment was defined as the latency time of the passive avoidance test. Whenever the rats did not enter the dark compartment within 180 seconds, the latency was recorded as 180 seconds.
Novel object recognition test was done (modified) according to a published procedure by Adeniyi et al. [28]. Briefly, each rat from the respective groups was exposed to two tests (T1 and T2) for 5 minutes each. The duration between T1 and T2 was 120 minutes. In T1 the rats were freely permitted to explore two identical objects for 5 minutes following which the rats were returned to their respective cages. After an inter-trial interval of 120 minutes, the rats were returned to the testing area for the second test T2 (5 minutes). In T2, one of the familiar old objects was replaced by a new object (novel object). The rats explored the objects for 5 minutes while an observer blinded to the rats' groupings recorded the time spent exploring the old and novel objects respectively. The memory index was calculated according to the method of Adeniyi et al. (2016) [28] as:
The rats were deeply anesthetized with phenobarbital sodium and transcardially perfused, firstly with 50 ml phosphate buffered saline and secondly, with 4% paraformaldehyde in Tris buffer (pH 7.4) through the right cardiac ventricle and ascending aorta. The brains were then removed, post-fixed in 10% phosphate-buffered formalin for 24 hours. The mPFC tissues were embedded in paraffin wax and sectioned at 5-µm thickness on a microtome and prepared for Nissl staining and glial fibrillary acidic protein (GFAP) immunohistochemistry for routine light microscopy.
This was done according to the modified method of Dribben et al. [29]. Briefly, sections of the mPFC were mounted on gel-coated slides and were allowed to thoroughly dry. After drying, the slides were rinsed in two changes of distilled water for a total of 10 minutes and then placed in Cresyl violet for 15 minutes. The slides were then rinsed under running tap water for 5 minutes, dehydrated in graded alcohol, and cleared in two changes of xylene.
Immunohistochemical analysis of GFAP was performed on the sections of the mPFC. Five-µm-thick sections of the mPFC were fixed with 10% phosphate-buffered formalin for 6 hours. After blocking endogenous peroxidase, the sections were incubated with the primary antibodies, polyclonal anti-GFAP (Dako, Glostrup, Denmark) diluted 1:100. The peroxidase reaction was visualized using 0.03% DAB and 0.005% hydrogen peroxide. The immunostained sections were slightly counterstained with Cresyl violet, dehydrated, cleared, and mounted in DPX (Dako).
Digital images of the Cresyl violet stained and GFAP immune-stained sections of the mPFC processed for histochemical and immunohistochemical observations were captured using Zeiss Axioscope A1 with a camera scope (AxioCam MRc, Carl Zeiss MicroImaging GmbH, Gottingen, Germany) attached to a computer interface.
The cell counts of GFAP-positive glial cells in the mPFC was carried out on the GFAP immunostained sections counterstained with Cresyl violet stain. The cell counts in the respective brain sections were performed by an experienced histopathologist, who was blind to the experimental procedures. The total number of GFAP immunopositive cells in all groups were counted in five sections per animal, which was selected with 120-µm interval from 4.70 to 2.70 mm ventral and 4.70 to 2.70 mm dorsal to the bregma with reference to the Mouse Brain Atlas [30]. Descriptive statistics for continuous data is presented by mean±standard deviation. To evaluate the statistical differences between the study groups, the Kruskal-Wallis test was used for non-parametric data. The statistical analysis of data obtained in the study was performed using the one-way ANOVA test. A P-value of <0.05 was considered statistically significant.
In the Morris water maze test, the treatment with alcohol, morphine and the combination of morphine and alcohol groups shows increased in escaped latency time when compared with the vehicle group. The escaped latency time are more pronounced in the combination of morphine and alcohol group than in the morphine or alcohol only groups (Fig. 2).
In the passive avoidance test, the treatment with alcohol, morphine and the combination of morphine and alcohol groups shows increased in escaped latency time when compared with the vehicle group. The escaped latency time are more pronounced in the combination of morphine and alcohol group than in the morphine or alcohol only groups (Fig. 3).
The vehicle group shows higher percentage of memory index when compared with the groups treated with alcohol, morphine and the combination of morphine and alcohol. However, the group that was treated with morphine alone shows high percentage memory index when compared with alcohol alone and the combined alcohol and morphine groups (Fig. 4).
In the vehicle treated rats, the Nissl-stained sections of the mPFC showed normal pyramidal neurons with no perineuronal vacuolation or cavitations, the Nissl substances were within the neurons. In the alcohol treated rats, the neurons were with features of chromatolysis, fragmented cytoplasm and peri-nuclear Nissl deposits, pyknotic neurons, and the neurons are with ruptured membrane. In the morphine treated group, Nissl distribution in the neurons were altered. The neurons were with neurodegenerative features such as neuronal vacuolation or cavitations, irregular distribution of Nissl's substances and chromatolysis, fragmented cytoplasm, and peri-nuclear Nissl deposits (Fig. 5A). These observations agree with the number of normal neuronal count in the mPFC of the treated rats from the respective groups (Fig. 5B).
GFAP immunopositive cells were observed in the mPFC of the experimental rats. GFAP immunopositive expression was observed in the section of the mPFC from the vehicle treated rats but no astrocytic scar was seen. However, the expression of GFAP immunopositivity in the mPFC section obtained from the alcohol-treated group was remarkably increased with astrocytic glial scars compared with the vehicle. The morphine treated group showed a marked increase of GFAP immunopositive expression of astrocytes with increased astrocytic density and prominent astroglial scar (black circle in Fig. 6A). In the morphine+alcohol treated group, there was a large number of neuronal loss with vacuolations; each of these vacuolated neurons are surrounded by deeply GFAP immunopositive astrocytes. The section is also characterized by patches of glia scar (Fig. 6A). These outcome is in consonant with the quantitative astrocytic cell count in the mPFC of the treated rats from the respective groups as there are less number of positive glial cells GFAP stained in the medial prefrontal cortex of the vehicle group compared to the alcohol, morphine and the combination of morphine and alcohol groups. Meanwhile, the combination of alcohol and morphine group shows a marked increase in the number of GFAP-positive glial cells when compared with the alcohol and morphine only groups (Fig. 6B).
Cognitive and memory impairments following exposure to opioids [31] or alcohol [11323334] in the animal model of drug addiction have been well documented by several behavioural paradigms used in evaluating cognition, learning, and memory.
In this study, we examined the effects of alcohol, morphine and the combination of morphine and alcohol on some cognitive behavioural parameters (using Morris water maze, passive avoidance test, and novel object recognition test) and subcellular pathological procedures (using histochemical and immunohistochemical protocol) in the mPFC of juvenile male rats. The observations made from the Morris water maze, passive avoidance, and novel object recognition tests showed that alcohol, morphine, and morphine+alcohol treatment significantly impair cognitive functions in juvenile male rats. These observations are suggestive of the deleterious hyper interaction between opioid receptors and alcohol resulting in the impaired plasticity of cognitive processes thereby potentiating the disruption of consolidatory processes associated with learning and memory and other executive cognitive dysfunctions with specific vulnerability to drug addiction. It is evident from previous studies that most of the substances abused can confer deleterious effects on brain structures and topographies associated with cognitive functions. In most instances, these deleterious effects seem to alter brain circuits associated with important aspects of cognition, such as learning and memory, attention, risk-taking, and motivation [35]. The neuropathological features seen in the mPFC of the treated rats further substantiate the behavioural deviations. There are controversial reports on the neurodegenerative [3637383940] or neuroprotective effects of morphine [41424344]. The pieces of evidence from our study agree with previously published reports on the neurodegenerative effects of morphine. Morphine-alcohol further aggravates neuropathological deviations in the mPFC of the treated rats. This is suggestive of the combined effect of the two substances in rapidly activating an interplay between the apoptosis and necrosis pathways thereby contributing to the neurodegenerative features seen in the CFV stained sections of the mPFC.
GFAP is the major protein expressed by the glial intermediate filaments in astrocytes. It has been recommended as a specific marker for evaluating and/or measuring the responses of astrocytes to several injuries in the CNS. An increase in GFAP expression is a cardinal feature of many pathological conditions of the CNS and astrocytes.
Astrocytes perform significant roles in the functional regulation of defence against oxidative and toxic insults around the neurons and synapses in the CNS microenvironment [4546]. Astrocyte activation has been reported to be initiated by environmental toxicant [47] and may experience a morphological modification with short or non-existent processes to permit phagocytosis [48]. Upregulation of glial cells has also been associated with the pathogenesis of plaques in many neurodegenerative conditions [49]. Hence, we presume that in the present study, upregulation of glial cells both in the cell count and in the expression of GFAP in the mPFC section could be suggestive of neurodegenerative effects induced by morphine and alcohol.
The administration of alcohol, morphine, and morphine+alcohol give rise to neuronal distortion and neuronal loss characterized by loss of cytoplasmic content, peripheral deposition of Nissl bodies, chromatolysis, neuronal vacuolation in the mPFC of the treated rats. These features are suggestive of the deterioration of cellular functions which ultimately leads to the destruction of cellular structures and cell death [50]. This agrees with the study of [5152]. The neurodegenerative effects of alcohol, morphine, and morphine+alcohol suggest that these substances induce their respective degenerative effects through reactive oxygen species associated oxidative stress which could have elicited the activated astroglial reaction, and neuronal cell death. There was no significant difference in the quantitative expression of GFAP immunopositive glial cell counts between the alcohol-treated and morphine-treated groups; however, in the morphine+alcohol group, a significant increase in the quantitative expression of GFAP immunopositive glial cell counts was observed relative to the alcohol-treated, morphine-treated, and vehicle-treated groups respectively. The number of GFAP immunopositive astrocytes and the complexity of the processes may also be related to the extent of the neural injury. The significant increase in the quantitative number of GFAP immunopositive astrocytes in the morphine+alcohol group in comparison with the alcohol, morphine, and vehicle-treated groups suggest that some essential and integral inflammatory pathway might have been selectively turned on in the biological make-up of the astrocytes.
Previous studies have shown that in the CNS, morphine or alcohol can alter the functional integrity of several physiological processes, oxidative impairment of cellular functions, alterations of membrane integrities, dysregulation of cell-cell interactions and signaling, and inhibitory or hyper-excitatory release of neurotransmitters [5152].
In this study, we observed a marked degeneration of neuronal cells in the mPFC of the treated rats vis-à-vis the vehicle. The mPFC plays a significant role in motivating and organizing addiction-related behaviour, and any form of lesion or modification in the function(s) of the mPFC may be interrelated with a marked predisposition to addictive behaviours [535455]. Exposure to alcohol or opioids have been reported to cause partial inactivation and lesion of the PFC and these alterations have been linked to neuronal loss and impairment behavioural sensitization [5657585960616263]. Therefore, we suggest that the marked neuronal loss observed in the mPFC could possibly account for the respective levels of cognitive deficit in the rats in the alcohol-treated, morphine-treated groups compared with the vehicle-treated group.
In conclusion, this study has shown the juvenile exposure to alcohol, morphine, and morphine+alcohol induces severe neuronal and glial cell alterations in the mPFC. This could possibly occur because of the presumably oxidative assault which could have triggered these cellular damages in the cytoarchitectural profile of the mPFC of the experimental rats. We also observed marked cognitive deficit following exposure to alcohol, morphine, and morphine+alcohol, however, the present study shows that morphine+alcohol treatment induces a significant adverse effect on all studied parameters compared with the alcohol, morphine, and vehicle-treated groups respectively.
Figures and Tables
Fig. 2
The latency of time of Morris water maze test across the groups (n=5 per group at P<0.001). a)Significant difference between vehicle and the other groups. b)Significant difference between alcohol, morphine, and morphine+alcohol groups. c)Significance difference between morphine and morphine+alcohol group.
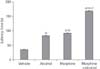
Fig. 3
The latency of time of passive avoidance test across the groups (n=5 per group at P<0.001). a)Significant difference between vehicle and the other groups. b)Significant difference between alcohol, morphine, and morphine+alcohol groups. c)Significance difference between morphine and morphine+alcohol group.
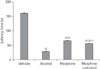
Fig. 4
The memory index of novel object recognition test across the groups (n=5 per group at P<0.001). a)Significant difference between vehicle and the other groups. b)Significant difference between alcohol, morphine, and morphine+alcohol groups. c)Significance difference between morphine and morphine+alcohol group.
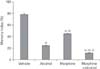
Fig. 5
(A) Photomicrographs of the medial prefrontal cortex of the representative rats in the vehicle, alcohol, morphine, and morphine+alcohol treated groups, respectively. In the vehicle group, the neurons are with intact cytoplasmic contents devoid of perineuronal vacuolation or cavitation, and the Nissl substances are well preserved; in the alcohol treated group, the neurons are with features of chromatolysis, fragmented cytoplasm and peri-nuclear Nissl deposits, pyknotic neurons, neurons with ruptured membrane; in the morphine treated group, Nissl distribution in the neurons are altered. The neurons are with neurodegenerative features such as; neuronal vacuolation, irregular distribution of Nissl's substances and chromatolysis, and peri-nuclear Nissl deposits, vascular structure; in the morphine+alcohol treated group, the neurons have condensed nuclei with nuclear karyorrhectic with concurrent swelling of neuronal dendrites (Cresyl violet staining, ×40). (B) Effect of treatments on the number of normal neurons in the medial prefrontal cortex obtained from the cresyl fast violet stained section (n=5 per group at P<0.001). a)Significant difference between vehicle and the other groups. b)Significant difference between alcohol, morphine and morphine+alcohol groups while. c)Significance difference between morphine and morphine+alcohol group.
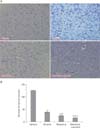
Fig. 6
(A) Anti–glial fibrillary acidic protein (GFAP) antibody labelled sections of the medial prefrontal cortex (mPFC) of the representative rats in the vehicle, alcohol, morphine, and morphine+alcohol treated groups respectively. There was a uniform distribution of GFAP immunopositive reactivity in the mPFC of the rat in the vehicle group showing astrocyte with normal cytological features. In the alcohol treated group, there were more GFAP immunopositive astrocytes with intensively stained cell bodies and elongated astrogliotic processes with scar formation (black circles). In the morphine treated group, few astrocytic densities were observed with prominent astroglial scar (black circle); few of the visible neurons are sparsely stained. In the morphine+alcohol treated group, there were many neurons with vacuolations; each of these vacuolated neurons are surrounded by GFAP immunopositive astrocytes; the section is also characterized by obvious patches of glia scars. Note: vascular structures (GFAP, ×40). (B) The graphical representative of the number of GFAP positive of glial cell in mPFC (n=5 per group at P<0.001). a)Significant difference between vehicle and the other groups. b)Significant difference between alcohol, morphine and morphine+alcohol groups while. c)Significance difference between morphine and morphine+alcohol group.
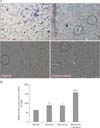
References
1. Kopnisky KL, Hyman SE. Molecular and cellular biology of addiction. In : Davis KL, Charney D, Coyle JT, Nemeroff C, editors. Neuropsychopharmacology: The Fifth Generation of Progress. Brentwood, TN: merican College of Neuropsychopharmacology;2002. p. 1367–1379.
2. Bohn LM, Gainetdinov RR, Lin FT, Lefkowitz RJ, Caron MG. Mu-opioid receptor desensitization by beta-arrestin-2 determines morphine tolerance but not dependence. Nature. 2000; 408:720–723.
3. Al-Hasani R, Bruchas MR. Molecular mechanisms of opioid receptor-dependent signaling and behavior. Anesthesiology. 2011; 115:1363–1381.


4. Martini L, Whistler JL. The role of mu opioid receptor desensitization and endocytosis in morphine tolerance and dependence. Curr Opin Neurobiol. 2007; 17:556–564.


5. Dumbili E. Changing patterns of alcohol consumption in Nigeria: an exploration of responsible factors and consequences. Med Soc Online. 2013; 7:20–33.
6. Guerri C, Bazinet A, Riley EP. Foetal alcohol spectrum disorders and alterations in brain and behaviour. Alcohol Alcohol. 2009; 44:108–114.


7. Wilhelm CJ, Guizzetti M. Fetal alcohol spectrum disorders: an overview from the glia perspective. Front Integr Neurosci. 2016; 9:65.


8. Giedd JN. Structural magnetic resonance imaging of the adolescent brain. Ann N Y Acad Sci. 2004; 1021:77–85.


9. Durston S, Davidson MC, Tottenham N, Galvan A, Spicer J, Fossella JA, Casey BJ. A shift from diffuse to focal cortical activity with development. Dev Sci. 2006; 9:1–8.


10. Counotte DS, Smit AB, Pattij T, Spijker S. Development of the motivational system during adolescence, and its sensitivity to disruption by nicotine. Dev Cogn Neurosci. 2011; 1:430–443.


11. Guerri C, Pascual M. Mechanisms involved in the neurotoxic, cognitive, and neurobehavioral effects of alcohol consumption during adolescence. Alcohol. 2010; 44:15–26.


12. Alfonso-Loeches S, Guerri C. Molecular and behavioral aspects of the actions of alcohol on the adult and developing brain. Crit Rev Clin Lab Sci. 2011; 48:19–47.


13. Crews FT, Braun CJ, Hoplight B, Switzer RC 3rd, Knapp DJ. Binge ethanol consumption causes differential brain damage in young adolescent rats compared with adult rats. Alcohol Clin Exp Res. 2000; 24:1712–1723.


14. Pascual M, Blanco AM, Cauli O, Miñarro J, Guerri C. Intermittent ethanol exposure induces inflammatory brain damage and causes long-term behavioural alterations in adolescent rats. Eur J Neurosci. 2007; 25:541–550.


15. Attwood AS, Munafò MR. Effects of acute alcohol consumption and processing of emotion in faces: implications for understanding alcohol-related aggression. J Psychopharmacol. 2014; 28:719–732.


16. Kolb B, Mychasiuk R, Muhammad A, Li Y, Frost DO, Gibb R. Experience and the developing prefrontal cortex. Proc Natl Acad Sci U S A. 2012; 109:Suppl 2. 17186–17193.


17. Holroyd CB, Coles MG, Nieuwenhuis S. Medial prefrontal cortex and error potentials. Science. 2002; 296:1610–1611.


18. Botvinick MM, Cohen JD, Carter CS. Conflict monitoring and anterior cingulate cortex: an update. Trends Cogn Sci. 2004; 8:539–546.


19. Ridderinkhof KR, Ullsperger M, Crone EA, Nieuwenhuis S. The role of the medial frontal cortex in cognitive control. Science. 2004; 306:443–447.


20. Bechara A, Damasio AR. The somatic marker hypothesis: a neural theory of economic decision. Games Econ Behav. 2005; 52:336–372.


21. Posner MI, Rothbart MK, Sheese BE, Tang Y. The anterior cingulate gyrus and the mechanism of self-regulation. Cogn Affect Behav Neurosci. 2007; 7:391–395.


22. Rushworth MF, Noonan MP, Boorman ED, Walton ME, Behrens TE. Frontal cortex and reward-guided learning and decision-making. Neuron. 2011; 70:1054–1069.


23. Euston DR, Gruber AJ, McNaughton BL. The role of medial prefrontal cortex in memory and decision making. Neuron. 2012; 76:1057–1070.


24. Shekarchizadeh H, Khami MR, Mohebbi SZ, Ekhtiari H, Virtanen JI. Oral health of drug abusers: a review of health effects and care. Iran J Public Health. 2013; 42:929–940.
25. Park JH, Choi HY, Cho JH, Kim IH, Lee TK, Lee JC, Won MH, Chen BH, Shin BN, Ahn JH, Tae HJ, Choi JH, Chung JY, Lee CH, Cho JH, Kang IJ, Kim JD. Effects of chronic scopolamine treatment on cognitive impairments and myelin basic protein expression in the mouse hippocampus. J Mol Neurosci. 2016; 59:579–589.


26. Lee JC, Park JH, Ahn JH, Kim IH, Cho JH, Choi JH, Yoo KY, Lee CH, Hwang IK, Cho JH, Kwon YG, Kim YM, Kang IJ, Won MH. New GABAergic neurogenesis in the hippocampal CA1 region of a gerbil model of long-term survival after transient cerebral ischemic injury. Brain Pathol. 2016; 26:581–592.


27. Kim YH, Park JH. Vanillin and 4-hydroxybenzyl alcohol attenuate cognitive impairment and the reduction of cell proliferation and neuroblast differentiation in the dentate gyrus in a mouse model of scopolamine-induced amnesia. Anat Cell Biol. 2017; 50:143–151.


28. Adeniyi PA, Ishola AO, Laoye BJ, Olatunji BP, Bankole OO, Shallie PD, Ogundele OM. Neural and behavioural changes in male periadolescent mice after prolonged nicotine-MDMA treatment. Metab Brain Dis. 2016; 31:93–107.


29. Dribben WH, Creeley CE, Farber N. Low-level lead exposure triggers neuronal apoptosis in the developing mouse brain. Neurotoxicol Teratol. 2011; 33:473–480.


30. Paxinos G, Franklin K. Paxinos and Franklin's the mouse brain in stereotaxic coordinates. 4th ed. San Diego, CA: Academic Press;2012.
31. Ma MX, Chen YM, He J, Zeng T, Wang JH. Effects of morphine and its withdrawal on Y-maze spatial recognition memory in mice. Neuroscience. 2007; 147:1059–1065.


32. Cippitelli A, Zook M, Bell L, Damadzic R, Eskay RL, Schwandt M, Heilig M. Reversibility of object recognition but not spatial memory impairment following binge-like alcohol exposure in rats. Neurobiol Learn Mem. 2010; 94:538–546.


33. Vetreno RP, Hall JM, Savage LM. Alcohol-related amnesia and dementia: animal models have revealed the contributions of different etiological factors on neuropathology, neurochemical dysfunction and cognitive impairment. Neurobiol Learn Mem. 2011; 96:596–608.


34. Swartzwelder HS, Acheson SK, Miller KM, Sexton HG, Liu W, Crews FT, Risher ML. Adolescent intermittent alcohol exposure: deficits in object recognition memory and forebrain cholinergic markers. PLoS One. 2015; 10:e0140042.


35. Nyberg F. Cognitive impairments in drug addicts. In : Gonzalez-Quevedo A, editor. Brain Damage: Bridging between Basic Research and Clinics. Rijeka: InTech;2012. p. 221–244.
36. Hodgson PS, Neal JM, Pollock JE, Liu SS. The neurotoxicity of drugs given intrathecally (spinal). Anesth Analg. 1999; 88:797–809.


37. Boronat MA, García-Fuster MJ, García-Sevilla JA. Chronic morphine induces up-regulation of the pro-apoptotic Fas receptor and down-regulation of the anti-apoptotic Bcl-2 oncoprotein in rat brain. Br J Pharmacol. 2001; 134:1263–1270.


38. Mao J, Sung B, Ji RR, Lim G. Neuronal apoptosis associated with morphine tolerance: evidence for an opioid-induced neurotoxic mechanism. J Neurosci. 2002; 22:7650–7661.


39. Atici S, Cinel L, Cinel I, Doruk N, Aktekin M, Akca A, Camdeviren H, Oral U. Opioid neurotoxicity: comparison of morphine and tramadol in an experimental rat model. Int J Neurosci. 2004; 114:1001–1011.


40. Turchan-Cholewo J, Liu Y, Gartner S, Reid R, Jie C, Peng X, Chen KC, Chauhan A, Haughey N, Cutler R, Mattson MP, Pardo C, Conant K, Sacktor N, McArthur JC, Hauser KF, Gairola C, Nath A. Increased vulnerability of ApoE4 neurons to HIV proteins and opiates: protection by diosgenin and L-deprenyl. Neurobiol Dis. 2006; 23:109–119.


41. Zhang W, Hong JS, Kim HC, Zhang W, Block ML. Morphinan neuroprotection: new insight into the therapy of neurodegeneration. Crit Rev Neurobiol. 2004; 16:271–302.


42. Peart JN, Gross ER, Gross GJ. Opioid-induced preconditioning: recent advances and future perspectives. Vascul Pharmacol. 2005; 42:211–218.


43. Rambhia S, Mantione KJ, Stefano GB, Cadet P. Morphine modulation of the ubiquitin-proteasome complex is neuroprotective. Med Sci Monit. 2005; 11:BR386–BR396.
44. Bekheet SH, Saker SA, Abdel-Kader AM, Younis AE. Histopathological and biochemical changes of morphine sulphate administration on the cerebellum of albino rats. Tissue Cell. 2010; 42:165–175.


46. Heller JP, Rusakov DA. Morphological plasticity of astroglia: understanding synaptic microenvironment. Glia. 2015; 63:2133–2151.


47. Block ML, Hong JS. Chronic microglial activation and progressive dopaminergic neurotoxicity. Biochem Soc Trans. 2007; 35(Pt 5):1127–1132.


48. Tremblay ME, Lowery RL, Majewska AK. Microglial interactions with synapses are modulated by visual experience. PLoS Biol. 2010; 8:e1000527.


49. Serrano-Pozo A, Frosch MP, Masliah E, Hyman BT. Neuropathological alterations in Alzheimer disease. Cold Spring Harb Perspect Med. 2011; 1:a006189.


50. Riancho J, Ruiz-Soto M, Villagrá NT, Berciano J, Berciano MT, Lafarga M. Compensatory motor neuron response to chromatolysis in the murine hSOD1(G93A) model of amyotrophic lateral sclerosis. Front Cell Neurosci. 2014; 8:346.


51. Liu LW, Lu J, Wang XH, Fu SK, Li Q, Lin FQ. Neuronal apoptosis in morphine addiction and its molecular mechanism. Int J Clin Exp Med. 2013; 6:540–545.
52. Bajic D, Commons KG, Soriano SG. Morphine-enhanced apoptosis in selective brain regions of neonatal rats. Int J Dev Neurosci. 2013; 31:258–266.


53. Van den Oever MC, Spijker S, Smit AB, De Vries TJ. Prefrontal cortex plasticity mechanisms in drug seeking and relapse. Neurosci Biobehav Rev. 2010; 35:276–284.


54. Myers-Schulz B, Koenigs M. Functional anatomy of ventromedial prefrontal cortex: implications for mood and anxiety disorders. Mol Psychiatry. 2012; 17:132–141.


55. Rafati A, Noorafshan A, Torabi N. Stereological study of the effects of morphine consumption and abstinence on the number of the neurons and oligodendrocytes in medial prefrontal cortex of rats. Anat Cell Biol. 2013; 46:191–197.


56. Carlezon WA Jr, Nestler EJ. Elevated levels of GluR1 in the midbrain: a trigger for sensitization to drugs of abuse? Trends Neurosci. 2002; 25:610–615.


57. Mikkola JA, Janhunen S, Hyytiä P, Kiianmaa K, Ahtee L. Rotational behaviour in AA and ANA rats after repeated administration of morphine and cocaine. Pharmacol Biochem Behav. 2002; 73:697–702.


58. Ojanen S, Koistinen M, Bäckström P, Kankaanpää A, Tuomainen P, Hyytiä P, Kiianmaa K. Differential behavioural sensitization to intermittent morphine treatment in alcohol-preferring AA and alcohol-avoiding ANA rats: role of mesolimbic dopamine. Eur J Neurosci. 2003; 17:1655–1663.


59. Sanchis-Segura C, Pastor R, Aragon CM. Opposite effects of acute versus chronic naltrexone administration on ethanol-induced locomotion. Behav Brain Res. 2004; 153:61–67.


60. Kerns RT, Ravindranathan A, Hassan S, Cage MP, York T, Sikela JM, Williams RW, Miles MF. Ethanol-responsive brain region expression networks: implications for behavioral responses to acute ethanol in DBA/2J versus C57BL/6J mice. J Neurosci. 2005; 25:2255–2266.


61. Ojanen SP, Hyytia P, Kiianmaa K. Behavioral sensitization and voluntary ethanol drinking in alcohol-preferring AA rats exposed to different regimens of morphine treatment. Pharmacol Biochem Behav. 2005; 80:221–228.

