Abstract
BACKGROUND/OBJECTIVES
Gallus gallus domesticus (GD) is a natural mutant breed of chicken in Korea with an atypical characterization of melanin in its tissue. This study investigated the effects of melanin extracts of GD on osteoblast differentiation and inhibition of osteoclast formation.
MATERIALS/METHODS
The effects of the melanin extract of GD on human osteoblast MG-63 cell differentiation were examined by evaluating cell viability, osteoblast differentiation, and expression of osteoblast-specific transcription factors such as bone morphogenetic protein 2 (BMP-2), small mothers against decapentaplegic homologs 5 (SMAD5), runt-related transcription factor 2 (RUNX2), osteocalcin and type 1 collagen (COL-1) by reverse transcription-polymerase chain reaction and western blotting analysis. We investigated the inhibitory effect of melanin on the osteoclasts formation through tartrate-resistant acid phosphatase (TRAP) activity and TRAP stains in Raw 264.7 cell.
RESULTS
The melanin extract of GD was not cytotoxic to MG-63 cells at concentrations of 50-250 µg/mL. Alkaline phosphatase (ALP) activity and bone mineralization of melanin extract-treated cells increased in a dose-dependent manner from 50 to 250 µg/mL and were 149% and 129% at 250 µg/mL concentration, respectively (P < 0.05). The levels of BMP-2, osteocalcin, and COL-1 gene expression were significantly upregulated by 1.72-, 4.44-, and 2.12-fold in melanin-treated cells than in the control cells (P < 0.05). The levels of RUNX2 and SMAD5 proteins were higher in melanin-treated cells than in control vehicle-treated cells. The melanin extract attenuated the formation of receptor activator of nuclear factor kappa-B ligand-induced TRAP-positive multinucleated RAW 264.7 cells by 22%, and was 77% cytotoxic to RAW 264.7 macrophages at a concentration of 500 µg/mL.
CONCLUSIONS
This study provides evidence that the melanin extract promoted osteoblast differentiation by activating BMP/SMADs/RUNX2 signaling and regulating transcription of osteogenic genes such as ALP, type I collagen, and osteocalcin. These results suggest that the effective osteoblastic differentiation induced by melanin extract from GD makes it potentially useful in maintaining bone health.
Osteoporosis, which is a metabolic condition characterized by decreased bone mass and increased risk of fracture, occurs frequently in women following menopause [1]. The osteoporosis in woman over the age of 60 years is a major bone disorder and is characterized by the reduction of endogenous estrogen hormone [1]. In addition, osteoporosis-induced bone frailty is due to excess osteoclastic activity and osteoblast repression, which lead to an imbalance in bone formation with bone resorption [2]. Therefore, maintaining the balance between osteoblasts and osteoclasts is an important strategy for bone health. Osteoblasts play a crucial role in bone regeneration through differentiation and proliferation [3]. Osteoblast differentiation appears marked strength and rigidity on bone while still maintaining some degree of elasticity [3].
The osteoclast cell is a tissue-specific macrophage polykaryon formed by differentiation induced by a tumor necrosis factor (TNF) family cytokine and monocyte/macrophage precursor cells at or near the bone surface [2]. The receptor activator of nuclear factor kappa-B ligand (RANKL), as osteoclast differentiation marker, enables the reconstitution of dynamic differentiation processes including cell fusion and activation of resorption by mature osteoclasts [2]. Dietary approaches can be used as alternative therapeutic strategies to slow the rate of bone loss. Numerous common foods contain naturally occurring bioactive molecules including bisphosphonates, calcitonin, phytoestrogens, vitamin D analogs, ipriflavone, and isoflavone, which have potential roles in preventing bone resorption and enhancing bone formation [4567].
Gallus gallus domesticus (GD), also known as Yeonsan Ogolgye, is a natural mutant breed of chicken found in Korea. It possesses unique morphological features such as black fluffy head feathers, earlobes, pupils, and feathers, and four toes [8]. Ogolgye chicken is a protein-rich food with a low fat content and is mainly used as a health food for pregnant women and infirmed individuals [9]. In addition, previous studies of the ogolgye chicken reported its physicochemical characteristics [8] and storage and sensory properties [10]. Ogolgye chicken was reported to efficaciously promote the formation of red blood cells in the bone marrow, and it showed medical effect such as in treating anemia and bleeding as well as antioxidant, antihypertensive [11], anti-inflammatory, and analgesic properties [12]. In our previous study, the water extracts of GD promoted alkaline phosphatase (ALP) activity and bone mineralization, and inhibited bone resorption [13]. However, studies investigating the effects of melanin extracted from GD on bone formation via osteoblast differentiation and inhibition of osteoclast effect have not been reported. Furthermore, to the best of our knowledge, there are no reports on the beneficial effects of GD on bone regeneration or differentiation.
Therefore, for the first time, this present study investigated the effect of melanin extracts of GD skin on osteoblast differentiation. Further, the potential bone-strengthening effect of melanin was examined by evaluating the effects of GD melanin on osteoblastic MG-63 cell differentiation. Melanin extracts of GD may increase ALP activity and bone mineralization. Furthermore, the positive effects of GD melanin on bone morphogenetic protein-2 (BMP-2), osteocalcin, type 1 collagen (COL-1), runt-related transcription factor 2 (RUNX2), and small mothers against decapentaplegic homologs 5 (SMAD5) as important biomarkers of bone formation and remodeling were investigated. Finally, the results may provide new insights into the osteoblastic differentiation induced by GD melanin as well as confirm its possible utilization as a functional food and bone health supplement.
GDs were obtained from Jisan Plantation (Chungnam, Korea) and reared until they were 3-year-old. Melanin was extracted from GD skin as described by Harki et al. [14]. Samples (200 g) were homogenized with alkali (1 M sodium hydroxide [NaOH], 200 mL at 110℃) for 30 min using a waring blender. After filtration, the dark-brown filtrate was acidified to pH 2.5 with 2 M hydrochloric acid (HCl) at room temperature for 2 h. The precipitate was collected by centrifugation (12,000 rpm for 30 min) and washed with distilled water. The crude melanin extracts were hydrolyzed with 40 mL of 7 M HCl for 2 h at 100℃. The non-hydrolysable residue was collected by centrifugation (1,000 rpm for 10 min) and washed with 0.01 M HCl solution, followed by distilled water. The non-hydrolysable melanin was re-dissolved in 20 mL 1 M potassium hydroxide (KOH) and 8 mL chloroform was added, followed by 0.8 mL 1-butanol. The mixture was shaken for 30 min under nitrogen and centrifuged (6,000 rpm for 10 min). The chloroform phase was discarded, and the KOH, and chloroform treatment steps in the above procedure were repeated twice. Finally, the melanin extract was precipitated by acidification and washed with distilled water. The precipitate melanin was stored in a −80℃ freezer and was used for later experiments. Chemicals were used from Sigma-Aldrich (St Louis, MO, USA) unless otherwise noted.
The GD melanin extract was characterized using electron ionization mass spectrometry (EI-MS), Fourier transform infrared (FTIR) spectroscopy and carbon-13 nuclear magnetic resonance (13C-NMR) imaging. The EI-MS was measured using a Jeol JMS-600 W mass spectrometer (Agilent Technologies Inc, Santa Clara, CA, USA). Attenuated total reflectance (ATR)-FTIR mounted with a diamond ATR crystal was used for spectral acquisition. The spectrum was recorded at 400-4,000 cm−1 using a cary 630 FTIR spectrophotometer (Agilent Technologies Inc.). The 13C-NMR was measured using a Bruker Avance AV-500 spectrometer (Bruker BioSpin GmbH, Rheinstetten, Germany) at a frequency of 115.91 MHz and 1,024 scans. The purified melanin was dissolved in dimethyl sulfoxide (DMSO), and the chemical shifts are expressed in parts per million (ppm).
Human osteoblast cell (MG-63) and osteoclast cell (RAW 264.7) (Korean Cell Bank, Seoul, Korea) were cultured in Rosewell Park Memorial Institute (RPMI) medium or Dulbecco's modified Eagle's medium (DMEM) containing 10% Fetal bovine serum (FBS; Hyclone, Logan, UT, USA) (Hyclone, Logan, UT, USA) and 1% penicillin-streptomycin (GIBCO, Grand Island, NY, USA) in a 5% CO2 incubator at 37℃.
Cell viability of MG-63 and RAW 264.7 cells (5 × 104) were cultured in a 96-well plate, incubated for 24 h, and the cell viability was determined using the 3-(4,5-dimethylthiazol-2-yl)-2,5-diphenyltetrazolium bromide (MTT; Promega, Madison, WI, USA) assay as described previously [13]. Briefly, the cells were treated with various concentrations (50-500 µg/mL) of the melanin extract for 24 h. Then, the MTT solution was added, followed by incubation at 37℃ for 4 h. The supernatant was removed, the formazan crystals that had formed were dissolved in DMSO, and the absorbance of the resulting solution was measured at 540 nm using a UVM 340 microplate reader (Biochrom Asys, Cambridge, UK).
ALP activity was measured as the rate of p-nitrophenyl phosphate (p-NPP) hydrolysis. Cells (5 × 104) were cultured in 96-well plates, incubated for 24 h, and then treated with melanin at various concentrations in RPMI, followed by incubation for 96 h. The cells were lysed in 0.1% Triton X-100, incubated with p-NPP at 37℃ for 60 min, and then the ALP activity was determined based on the optical density (OD) values measured at 405 nm using a microplate reader.
The degree of mineralization was analyzed using alizarin red S staining after 14 days of melanin extract treatment. Briefly, The cells were washed with phosphate-buffered saline (PBS) and then fixed with 70% ethanol for 1 h and stained with 40 mM alizarin red S in deionized water (pH 4.2) for 15 min. After aspirating the alizarin red S solution, cells were incubated in PBS for 15 min on an orbital rotator and then rinsed once with fresh PBS, followed by destaining for 15 min with 10% (w/v) cetylpyridinium chloride in 10 mM sodium phosphate (pH 7.0). The extracted stain was transferred to a 96-well plate, and the absorbance was measured at 550 nm by a microplate reader.
RAW 264.7 cells (5 × 104) were cultured in 96-well plates containing DMEM with 10% FBS, and the medium was replaced with the melanin in differentiation medium containing 50 ng/mL RANKL, which was changed every 2 days. After 5 days, the medium was removed, the cell monolayer was gently washed twice using PBS, the cells were fixed in 3.5% formaldehyde for 10 min, and then they were washed with distilled water. Then, the cells were incubated in 50 mM citrate buffer (pH 4.5) containing 10 mM sodium tartrate and 6 mM p-NPP. After 1 h incubation, the mixtures were transferred to new 96-well plates containing an equal volume of 0.1 N NaOH, and the absorbance was measured at 405 nm by a microplate reader, and TRAP activity was expressed as the percentage of the untreated control. TRAP staining was performed as follows: after differentiating the RAW 264.7 cells into osteoclasts for 5 days, the medium was removed, and the cells were gently washed twice with PBS. The cells were fixed in 3.5% formaldehyde for 10 min and washed with distilled water. The cells were incubated at 37℃ for 1 h in a humid and light-protected incubator using a leukocyte acid phosphatase assay kit (Sigma), as directed by the manufacturer. The cells were washed with distilled water, and TRAP-positive multinucleated cells were observed under a light microscope.
We performed a RT-PCR with glyceraldehyde 3-phosphate dehydrogenase (GAPDH) as an internal control. RNA was isolated using TRIzol reagent (Thermo Fisher Scientific, Inc., Waltham, MA, USA). Aliquots (1 µg) of total RNA were reverse transcribed to cDNA using SuperScript III Reverse Transcriptase (Invitrogen, Carlsbad, CA, USA). Primer sequences were as follows: GAPDH: 5'
CAAGAAGGTGGTGAAGCAGG -3' (forward) and 5'-GATGGTACATGACAAGGTGC-3' (reverse), BMP-2: 5'-TCAT AAAACCTGCAACAGCCAACTCG-3' (forward) and 5'-GCTGTACTA GCGACACCCAC-3' (reverse), osteocalcin: 5'-GGCCAGGCAGGTGC GAAGC-3' (forward) and 5'-GCCAGGCCAGCAGAGCGACAC-3' (reverse), and COL-1: 5'-AGCGCTGGTTTCGACTTCAGCTTCC-3' (forward) and 5'-CATCGGCAGGGTCGGAGCCCT-3' (reverse). The PCR products were detected using 1.2% agarose/ethidium bromide gel electrophoresis and photographed.
The cells were homogenized with radioimmunoprecipitation assay buffer with protease inhibitor (Roche, Mannheim, Germany), and centrifuged at 13,000 rpm for 10 min at 4℃. The total protein contents were determined using a Bio-Rad protein kit (Bio-Rad, Hercules, CA, USA) with bovine serum albumin (BSA) as the standard. The protein samples were subjected to electrophoresis with sodium dodecyl sulfate-polyacrylamide gel electrophoresis and transferred onto polyvinylidene fluoride membranes, which were blocked with 5% BSA in Tris-buffered saline (TBS) containing Tween 20, followed by incubation with the appropriate specific primary antibodies: RUNX2 (Abcam, Cambridge, MA, USA), BMP-2 (Abcam), SMAD5 (Santa Cruz Biotechnology, Santa Cruz, CA, USA) and β-actin (Cell Signaling Technology, Danvers, MA, USA). This was followed by incubation with the secondary goat anti-rabbit IgG (H+L) horseradish peroxidase (HRP) conjugate (Zymax, San Francisco, CA, USA) or goat anti-mouse IgG (H+L) HRP conjugate (ZyMax) antibodies. The antigen-antibody complexes were visualized using enhanced chemiluminescence. Densitometry analysis of the blotted membranes was performed using a C-DiGit Blot Scanner (Li-COR, Lincoln, NE, USA).
The data are showed as the mean ± SD of triplicate experiments. Statistical analyses were analyzed by the statistical package for the social sciences (SPSS) version 18.0 (SPSS Inc., Chicago, IL, USA). The groups were compared using a one-way analysis of variance (ANOVA) followed by post hoc Duncan's multiple range tests and P-values < 0.05 were considered statistically significant.
As shown in Fig. 1A, the EI-MS spectrum of melanin extracted from GD skin showed an ion peak at m/z 575. This data led to the identification of the compound as the trimer, melanin. The characteristic properties of the EI-MS spectrum of melanin were similar to those of melanin extracted from wild turkey were [15]. This is the first report of the trimer melanin in GD skin. The FTIR spectroscopy result (Fig. 1B) showed the melanin peak at 2,922 cm−1, which indicates C-H stretching. Absorption at 1,625 cm−1 was attributed to aromatic ring C=C stretching. The FTIR spectrum of this pigment was similar to that of melanin purified from Klebsiella sp. GSK and Shewanella algae BrY [1617].
As shown in Fig. 1C, the 13C-NMR spectrum of GD melanin displayed high intensity in the region of aromatic fragments (0-50 ppm). Peaks in the region of 10-45 ppm were assigned to the carbon atoms of methyl (such as CH3CH2 and CH3COO) and methylene (-CH2-) groups. Resonance peaks at 55-60 ppm were attributable to the carbon atoms in the CH-N or CH-S groups. The 13C-NMR spectrum of this pigment was similar to that of melanin purified from Lachnum YM404 [18]. Thus, the results of EI-MS, FTIR, and 13C-NMR confirmed the structure of the black pigment as melanin.
The effect of various concentrations of melanin on the proliferation of MG-63 cells was investigated using the MTT assay. At concentrations of 50, 150, and 250 µg/mL, melanin was not toxic to MG-63 cells (Fig. 2A). We examined the effect of melanin on ALP activity during osteoblast differentiation of MG-63 cells. As shown in Fig. 2B, melanin-treated cells exhibited significantly increased ALP activity, and the effect was concentration-dependent from 50-250 µg/mL (Fig. 2B) (P < 0.05). At 50 µg/mL, the cellular ALP activity of melanin extract increased 127% as compared to that observed in the control cells. At 250 µg/mL, the cellular ALP activity, especially, was increased by 1.5-fold compared to that observed in the control cells. These findings suggest that melanin stimulated osteoblast differentiation.
The effect of melanin on mineralization in MG-63 cells was investigated using alizarin red S staining and quantified using calcium deposition analysis. As shown in Fig. 3A, the bone nodules formed in the melanin-treated cells exhibited a clearer red color than those in the control cells. The 250 µg/mL melanin-treated cells exhibited an increase in the mineralization level. Treatment with melanin dose-dependently increased bone nodule formation in MG-63 cells than in the control cells (Fig. 3B).
The intercellular mineralization level was higher and the effect was concentration-dependent (Fig. 3B). The degree of bone mineralization was the highest at 129% when treated with 250 µg/mL melanin extract compared to that reported for the control. Thus, melanin effectively accelerated osteoblast maturation, which enhanced bone mineralization of MG-63 cells.
Before evaluating whether melanin could suppress osteoclast differentiation, we needed to confirm that it was not cytotoxic in vitro. Therefore, we treated the cells with four different concentrations of melanin (0, 50, 100, 250, and 500 µg/mL) for 96 h to determine the doses that inhibited cell proliferation. In RAW 264.7 osteoclasts, the toxicity of melanin was observed at < 250 µg/mL concentration. Furthermore, melanin showed a cytotoxic effect against osteoclast cells at 500 µg/mL (Fig. 4A). As shown in Fig. 4B, the melanin extract inhibited TRAP activity to 78% (22% inhibition) relative to the control at 500 µg/mL (Fig. 4B). However, the TRAP activity was not inhibited at melanin concentrations ≤ 250 µg/mL. TRAP-positive multinucleated osteoclasts were observed using light microphotography (Fig. 4C). As shown in Fig. 4C, treatment with melanin reduced the number of multinucleated TRAP-positive cells.
After 96 h of GD melanin treatment, RT-PCR analysis showed significantly increased BMP-2, osteocalcin, and COL-1 gene expression (Fig. 5). The level of BMP-2 gene expression significantly increased by 1.72-fold compared with that of the untreated control group. Furthermore, osteocalcin and COL-1 expression significantly increased by 4.44- and 2.12-fold in treated cells compared with that of the control group, respectively. These results suggest that melanin promoted osteogenesis and mineralization as observed by analyses of osteoblast differentiation markers.
The level of BMP-2 and RUNX2 protein was significantly higher in cells treated with melanin than in those treated with the control vehicle (Fig. 6A and B). In addition, BMP signaling strongly induces osteoblast differentiation and bone formation via the transforming growth factor (TGF) superfamily, termed SMADs [1920]. SMAD1, SMAD5, and SMAD8 are activated by BMP signaling and stimulate osteoblast differentiation [1920]. The protein expression levels of SMAD5 increased gradually during osteoblast differentiation (Fig. 6A and B). The melanin extract treatment significantly increased SMAD5 levels on differentiation day 4 (Fig. 6).
The skin of the Ogolgye chicken has a characteristic black color, which is caused by the pigment melanin. In our previous study, we reported the effects of water extracts of GD flesh and skin on osteoblast differentiation and osteoclast inhibition. Our previous results showed that melanin significantly increased ALP activity and osteoblast mineralization and inhibited osteoclast formation [13]. Based on this experiment, we evaluated the effect of this extract on osteoblast differentiation. Furthermore, our result showed that the melanin extract enhanced proliferation and differentiation of osteoblastic cells via BMP-2/RUNX2/SMAD5 signal. These results suggest that the melanin extract modulates bone formation by regulating osteoblasts.
Melanin is a high-molecular-weight, black or brown colored pigment synthesized by the oxidative polymerization of indolic or phenolic compounds [21]. The melanin extract from silkie fowl skin had reducing and chelating power against Fe2+ [9]. A recent study reported the antioxidant activity of melanin from Aspergillus nidulans [21]. The present study is the first to report that melanin extract enhanced osteoblast differentiation and mineralization of MG-63 cells.
Our study demonstrated that melanin extract increased ALP activity as well as mineralization. In osteoporosis, osteoblastic proliferation and ALP activity decrease [222324]. As shown in Fig. 2, 50-250 µg/mL of the melanin extract increased MG-63 cell proliferation concentration-dependently. Thus, our results demonstrate that melanin activated MG-63 cell proliferation and differentiation. Furthermore, the extract was not cytotoxic against the differentiated MG-63 cells. Bone regeneration induced osteoblast proliferation, increased ALP activity, and stimulated intracellular calcium deposition, extracellular matrix formation, and mineralization [2223]. ALP is an important indicator of bone mineralization, an early biomarker for osteopenia, and is expressed by all osteoblastic cells [24]. The differentiated osteogenic cells exhibited increased ALP activity and enhanced bone mineralization [222324]. The present study showed that melanin significantly increased ALP activities concentration-dependently (Fig. 2B). These findings demonstrate that melanin enhanced osteoblast differentiation by upregulating the differentiation of MG-63 cells.
During mineralization, cells produce extracellular calcium deposits, which can be assessed using alizarin red S staining. The melanin-treated cells were incubated for 14 days, stained, and then quantified using alizarin red S and an ELISA plate reader. Our result provides convincing evidence that melanin efficiently induced mineralization of MG-63 cells according to dose-dependent increased (Fig. 3). Our results demonstrated that the ALP activity of melanin-treated cells was stimulated and the calcified nodules formed were stained a deep red color compared to those of the control cells.
In this study, the expression level of COL-1 and osteocalcin significantly increased after melanin treatment of MG-63 cells. Our results showed that the expression level of markers (BMP-2, RUNX2, osteocalcin, and COL-1) was upregulated in response to melanin treatment in cultured MG-63 cells. RT-PCR and Western blotting analysis showed that the expression level of BMP-2 increased 1.72-fold and 4.28-fold compared to that of the control differentiated MG-63 cells, respectively. These results confirmed the successful enhancement of the osteogenic differentiation of MG-63 cells. Which was mediated through BMP-induced collagen synthesis using melanin as a stimulator. In addition, the expression of RUNX2 and its down-regulated gene osteocalcin, which is a non-collagenous protein, was more markedly upregulated in extract-treated cells than in the control cells [2526]. In this regard, our western blotting and RT-PCR analysis of BMP-2, SMAD5, and RUNX2 genes confirmed that RUNX2 is the downstream molecular biomarker for melanin-induced BMP-2/SMAD5 osteogenic signaling. Previous studies showed that BMPs require extracellular collagen matrix to improve osteoblastic differentiation, mineralization, and osteogenic differentiation gene expression [222728]. RUNX2 needs BMP/SMADs signaling to induce osteoblast-specific gene expression [222930]. These results confirm that melanin promoted osteoblast differentiation and induced the BMP/SMADs/RUNX2 signaling pathway in differentiated MG-63 cells.
For the first time, the present study demonstrates the effect of melanin extract from GD on osteoblast differentiation and mineralization. Furthermore, our investigation indicates that the melanin extract from GD significantly induced osteoblast differentiation. Melanin stimulated ALP activity and increased the expression level of BMP-2, SMAD5, RUNX2, osteocalcin and COL-1 in osteoblastic cells. The findings of the current study provide proof of the positive effects of melanin on osteoblast differentiation and may help to delineate the potential role of melanin in the treatment of bone metabolism disorders. To evaluate the possible application of this extract as a bone health supplement, its bioavailability will be examined in future in vivo studies.
Figures and Tables
Fig. 1
Characterization of melanin extracted from Gallus gallus domesticus (GD) skin.
(A) Electron ionization mass spectrometry (EI-MS) spectra of melanin. (B) Attenuated total reflection (ATR)-Fourier transform infrared (FTIR) spectra of melanin. (C) Carbon-13 nuclear magnetic resonance (13C-NMR) of melanin.
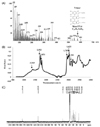
Fig. 2
Effect of melanin extracted from Gallus gallus domesticus (GD) on viability and alkaline phosphatase (ALP) activity of MG-63 osteoblast cells during differentiation.
Cells were treated with GD melanin at 0, 50, 150, and 250 µg/mL for 24 h, and 96 h for ALP activity. (A) Cell proliferation was measured using 3-(4,5-dimethylthiazol-2-yl)-2,5-diphenyltetrazolium bromide (MTT) assay. (B) ALP activity, as described in the materials and methods section. Values are mean ± SD (n = 3). Values with different letters were significantly different using Duncan's multiple range test (P < 0.05).
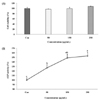
Fig. 3
Mineralization of MG-63 cells treated with melanin extracted from Gallus gallus domesticus (GD) determined using alizarin red S staining after 4 days of differentiation.
(A) Mineralized matrix was stained with alizarin red S. Melanin (250 µg/mL) significantly increased intracellular calcium. (B) Quantitation of mineralization using alizarin red S staining, as described in the materials and methods section. Values are mean ± SD (n = 3). Values with different letters were significantly different using Duncan's multiple range test (P < 0.05).
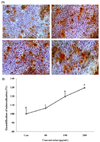
Fig. 4
Effect of melanin extracted from Gallus gallus domesticus (GD) on viability and tartrate-resistant acid phosphatase (TRAP) activity of RAW 264.7 cells during their differentiation.
Cells were treated with GD melanin at 0, 50, 150, 250 and 500 µg/mL for 2 days, and 4 days for TRAP activity. (A) Cell proliferation was measured using 3-(4,5-dimethylthiazol-2-yl)-2,5-diphenyltetrazolium bromide (MTT) assay. (B) TRAP activity, as described in materials and methods section. (C) TRAP staining using TRAP stain kit. Each value is mean ± SD (n = 3). Values with different letters were significantly different using Duncan's multiple range test (P < 0.05).
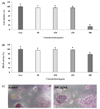
Fig. 5
Gene expression of bone morphogenetic protein-2 (BMP-2), osteocalcin and type 1 collagen (COL-1) using reverse transcription-polymerase chain reaction (RT-PCR).
Cells were differentiated for 4 days in differentiation medium in the presence or absence of melanin (250 µg/mL). (A) The mRNA expression of osteoblast differentiation factors such as BMP-2, COL-1, and osteocalcin. (B) Relative expression was quantified using image J program and calculated according to the reference bands of glyceraldehyde 3-phosphate dehydrogenase (GAPDH). Values are mean ± SD (n = 3). Values with different letters were significantly different using the Duncan's multiple range test (P < 0.05).
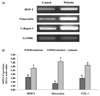
Fig. 6
Effects of melanin extracted from Gallus gallus domesticus (GD) on bone morphogenetic protein-2 (BMP) signal pathway in MG-63 cells.
Cells were differentiated for 4 days in differentiation medium in the presence or absence of melanin (250 µg/mL). (A) Protein expression of BMP signaling pathway-related markers such as BMP-2, runt-related transcription factor 2 (RUNX2), and small mothers against decapentaplegic homologs 5 (SMAD5) was detected by Western blot. (B) Relative expression was quantified by image J and calculated according to the reference bands of β-actin. Each value is expressed as mean ± SD (n = 3). Values with different letters were found to be significantly different using the Duncan's multiple range test (P < 0.05).
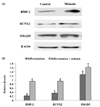
Notes
References
1. Vondracek SF, Linnebur SA. Diagnosis and management of osteoporosis in the older senior. Clin Interv Aging. 2009; 4:121–136.


2. Boyle WJ, Simonet WS, Lacey DL. Osteoclast differentiation and activation. Nature. 2003; 423:337–342.


3. Feng J, Shi Z, Ye Z. Effects of metabolites of the lignans enterolactone and enterodiol on osteoblastic differentiation of MG-63 cells. Biol Pharm Bull. 2008; 31:1067–1070.


4. Valero MA, Loinaz C, Larrodera L, Leon M, Moreno E, Hawkins F. Calcitonin and bisphosphonates treatment in bone loss after liver transplantation. Calcif Tissue Int. 1995; 57:15–19.


5. Kardinaal AF, Morton MS, Brüggemann-Rotgans IE, van Beresteijn EC. Phyto-oestrogen excretion and rate of bone loss in postmenopausal women. Eur J Clin Nutr. 1998; 52:850–855.


6. Richy F, Schacht E, Bruyere O, Ethgen O, Gourlay M, Reginster JY. Vitamin D analogs versus native vitamin D in preventing bone loss and osteoporosis-related fractures: a comparative meta-analysis. Calcif Tissue Int. 2005; 76:176–186.


7. Alekel DL, Germain AS, Peterson CT, Hanson KB, Stewart JW, Toda T. Isoflavone-rich soy protein isolate attenuates bone loss in the lumbar spine of perimenopausal women. Am J Clin Nutr. 2000; 72:844–852.


8. Cho CM, Park CK, Lee MY, Lew ID. Physicochemical characteristics of silky fowl (Gallus domesticus var. silkies). Korean J Food Sci Anim Resour. 2006; 26:306–314.
9. Lin LC, Chen WT. The study of antioxidant effects in melanins extracted from various tissues of animals. Asian-Australas J Anim Sci. 2005; 18:277–281.


10. Chae HS, An CN, Yoo YM, Park BY, Cho SH, Kim JH, Lee JM, Choi YI. Quality stability of high pressure boiled extract of ogol chicken during storage periods. Korea J Poult Sci. 2002; 29:279–286.
11. Liu JH. Study on antioxidation, anti-hypertension and hematopoiesis of taihe black-bone silky fowl (Gallus gallus domesticus brission) bioactive peptides [PhD thesis]. Nanchang: Nanchang University;2011.
12. Wang Y. Study on several functionalities of taihe black-bone silky fowl peptides [master's thesis]. Nanchang: Nanchang University;2011.
13. Yoo HS, Chung KH, Lee KJ, Kim DH, An JH. Effect of Gallus gallus var. domesticus (Yeonsan ogolgye) extracts on osteoblast differentiation and osteoclast formation. Microbiol Biotechnol Lett. 2015; 43:322–329.


14. Harki E, Talou T, Dargent R. Purification, characterisation and analysis of melanin extracted from Tuber melanosporum Vitt. Food Chem. 1997; 58:69–73.


15. Liu SY, Shawkey MD, Parkinson D, Troy TP, Ahmed M. Elucidation of the chemical composition of avian melanin. RSC Adv. 2014; 4:40396–40399.


16. Sajjan S, Kulkarni G, Yaligara V, Kyoung L, Karegoudar TB. Purification and physiochemical characterization of melanin pigment from Klebsiella sp. GSK. J Microbiol Biotechnol. 2010; 20:1513–1520.


17. Turick CE, Tisa LS, Caccavo F Jr. Melanin production and use as a soluble electron shuttle for Fe(III) oxide reduction and as a terminal electron acceptor by Shewanella algae BrY. Appl Environ Microbiol. 2002; 68:2436–2444.


18. Ye M, Guo GY, Lu Y, Song S, Wang HY, Yang L. Purification, structure and anti-radiation activity of melanin from Lachnum YM404. Int J Biol Macromol. 2014; 63:170–176.


19. Javed A, Bae JS, Afzal F, Gutierrez S, Pratap J, Zaidi SK, Lou Y, van Wijnen AJ, Stein JL, Stein GS, Lian JB. Structural coupling of Smad and Runx2 for execution of the BMP2 osteogenic signal. J Biol Chem. 2008; 283:8412–8422.


20. Javed A, Afzal F, Bae JS, Gutierrez S, Zaidi K, Pratap J, van Wijnen AJ, Stein JL, Stein GS, Lian JB. Specific residues of RUNX2 are obligatory for formation of BMP2-induced RUNX2-SMAD complex to promote osteoblast differentiation. Cells Tissues Organs. 2009; 189:133–137.


21. de Cássia R, Pombeiro-Sponchiado SR. Antioxidant activity of the melanin pigment extracted from Aspergillus nidulans. Biol Pharm Bull. 2005; 28:1129–1131.


22. Xia L, Yin Z, Mao L, Wang X, Liu J, Jiang X, Zhang Z, Lin K, Chang J, Fang B. Akermanite bioceramics promote osteogenesis, angiogenesis and suppress osteoclastogenesis for osteoporotic bone regeneration. Sci Rep. 2016; 6:22005.


23. Kim BS, Kang HJ, Park JY, Lee J. Fucoidan promotes osteoblast differentiation via JNK- and ERK-dependent BMP2-Smad 1/5/8 signaling in human mesenchymal stem cells. Exp Mol Med. 2015; 47:e128.


24. Jeon EJ, Lee DH, Kim YJ, Ahn J, Kim MJ, Hwang JT, Hur J, Kim M, Jang YJ, Ha TY, Seo DH, Lee JS, Sung MJ, Jung CH. Effects of yuja peel extract and its flavanones on osteopenia in ovariectomized rats and osteoblast differentiation. Mol Nutr Food Res. 2016; 60:2587–2601.


25. Pan W, Quarles LD, Song LH, Yu YH, Jiao C, Tang HB, Jiang CH, Deng HW, Li YJ, Zhou HH, Xiao ZS. Genistein stimulates the osteoblastic differentiation via NO/cGMP in bone marrow culture. J Cell Biochem. 2005; 94:307–316.


26. Komori T, Yagi H, Nomura S, Yamaguchi A, Sasaki K, Deguchi K, Shimizu Y, Bronson RT, Gao YH, Inada M, Sato M, Okamoto R, Kitamura Y, Yoshiki S, Kishimoto T. Targeted disruption of Cbfa1 results in a complete lack of bone formation owing to maturational arrest of osteoblasts. Cell. 1997; 89:755–764.


27. Xiao G, Gopalakrishnan R, Jiang D, Reith E, Benson MD, Franceschi RT. Bone morphogenetic proteins, extracellular matrix, and mitogen-activated protein kinase signaling pathways are required for osteoblast-specific gene expression and differentiation in MC3T3-E1 cells. J Bone Miner Res. 2002; 17:101–110.


28. Suzawa M, Takeuchi Y, Fukumoto S, Kato S, Ueno N, Miyazono K, Matsumoto T, Fujita T. Extracellular matrix-associated bone morphogenetic proteins are essential for differentiation of murine osteoblastic cells in vitro. Endocrinology. 1999; 140:2125–2133.


29. Lee KS, Kim HJ, Li QL, Chi XZ, Ueta C, Komori T, Wozney JM, Kim EG, Choi JY, Ryoo HM, Bae SC. Runx2 is a common target of transforming growth factor beta1 and bone morphogenetic protein 2, and cooperation between Runx2 and Smad5 induces osteoblastspecific gene expression in the pluripotent mesenchymal precursor cell line C2C12. Mol Cell Biol. 2000; 20:8783–8792.

