Abstract
Background
N-ras mutations are one of the most commonly detected abnormalities of myeloid origin. N-ras mutations result in a constitutively active N-ras protein that induces uncontrolled cell proliferation and inhibits apoptosis. We analyzed N-ras mutations in adult patients with AML at a particular institution and compared pyrosequencing analysis with a direct sequencing method for the detection of N-ras mutations.
Methods
We analyzed 90 bone marrow samples from 83 AML patients. We detected N-ras mutations in codons 12, 13, and 61 using the pyrosequencing method and subsequently confirmed all data by direct sequencing. Using these methods, we screened the N-ras mutation quantitatively and determined the incidence and characteristic of N-ras mutation.
Results
The incidence of N-ras mutation was 7.2% in adult AML patients. The patients with N-ras mutations showed significant higher hemoglobin levels (P=0.022) and an increased incidence of FLT3 mutations (P=0.003). We observed 3 cases with N-ras mutations in codon 12 (3.6%), 2 cases in codon 13 (2.4%), and 1 case in codon 61 (1.2%). All the mutations disappeared during chemotherapy.
Conclusions
There is a low incidence (7.2%) of N-ras mutations in AML patients compared with other populations. Similar data is obtained by both pyrosequencing and direct sequencing. This study showed the correlation between the N-ras mutation and the therapeutic response. However, pyrosequencing provides quantitative data and is useful for monitoring therapeutic responses.
The N-ras oncogene is a member of a family of membrane-associated proteins that instigate signal transduction upon ligand binding to a variety of membrane receptors. N-ras proteins regulate cell proliferation, differentiation, and apoptosis by cycling between active GTP-bound and inactive GDP-bound conformations [1, 2]. The most common mutations involve a single base substitution in codon 12, 13, or 61 and lead to constitutively active Ras. In its constitutively active, GTP-bound state, Ras induces uncontrolled cell proliferation and inhibits apoptosis [1, 2]. N-ras mutations have been identified in various solid tumors as well as hematologic malignancies [2]. The prognostic significance of N-ras mutations is still unclear. Some groups have proposed that Ras mutations are related to a better prognosis [3], whereas others have proposed that mutated Ras genes are associated with short survival [4], and many others have been unable to verify the clinical significance of Ras mutations [5-13].
Most recent studies examining the presence of Ras mutations in cancer cells have been performed using PCR followed by dideoxy DNA sequencing or conformation-based separation such as single strand conformation polymorphism (SSCP), denaturing gradient gel electrophoresis, restriction fragment length polymorphism (RFLP), or HPLC [6, 8-11]. However, these techniques are labor intensive and time consuming and are therefore not suitable for routine clinical practice. Furthermore, it has been reported that SSCP analysis gives false-negative results in approximately 33% of cases identified as positive [14]. Sanger sequencing is the most commonly used sequencing method; however, it does not detect some mutant sequences that are present in the background of abundant wild-type DNA sequences. Compared with dideoxy sequencing, pyrosequencing has greater analytical sensitivity for the detection of mutant DNA mixed with wild-type DNA. Pyrosequencing is a simple, real-time, non-electrophoretic, high-throughput mutation detection assay that can be applied to large clinical studies [15-17]. However, the practical aspects and efficiency of pyrosequencing for the analysis of N-ras mutations have not been evaluated in clinical settings.
The purpose of this study was to detect N-ras mutations in 83 Korean adult patients with AML and to compare the performance of a pyrosequencing kit to a direct sequencing method for the detection of N-ras mutations.
Eighty-three adult patients that attended the Hematology and Oncology Department, Gachon University Gil Medical Center, Incheon, Korea from May 2004 to February 2011 were enrolled to analyze the biological and prognostic impact of N-ras mutations. The median age of the study population was 55.0 yr (range: 20.0-88.0 yr). A large proportion (43.4%; 36 patients) of patients were older than 65 yr. The male-to-female ratio was 1.3:1 (47 men: 36 women). All patients were newly diagnosed with AML, except some patient with t(15;17) who were diagnosed with acute promyelocytic leukemia (APL). Informed consent for tissue collection and the research studies was approved by the Institutional Review Board of Gachon University Gil Medical Center. The patients were observed from May 2004 to January 2012. Bone marrow (BM) aspirates and biopsy specimen slides were classified into AML subtypes according to the WHO (2008) classifications [18]. The following clinical characteristics were analyzed at diagnosis: age, sex, hemoglobin, peripheral white blood cell (WBC) count, platelet count, serum lactate dehydrogenase (LDH) concentration, cytogenetic findings, and percentage of blasts in BM aspirates. After the induction and consolidation of chemotherapy, the patients were reevaluated for clinical and cytological findings of leukemia or treatment response. Complete remission (CR) was defined as a normocellular BM containing fewer than 5% blasts.
Of the 83 patients, 58 were treated with a standard protocol, 45 patients received only standard chemotherapy, and 13 patients were treated with standard chemotherapy as well as BM transplantation (BMT). Due to the physical condition of the older patients, 10 patients were given palliative low-dose 1-β-D-arabinofuranosylcytosine (Ara-C) treatment only. During the course of this study, 7 patients were transferred to other hospitals or were lost to follow-up therapies. Another 8 patients were denied therapy.
Chromosomal analysis was performed on the BM aspirates by G-banding. Karyotypes were designated according to the International System for Human Cytogenetic Nomenclature (2009) [19].
RNA from the BM aspirates was extracted using the Qiagen RNeasy Mini kit (Qiagen, Valencia, CA, USA) according to the manufacturer's instructions. After reverse transcription PCR using the Pure Extreme kit (MBI Fermentas, St Leon-Rot, Germany), gene rearrangements were analyzed using Seeplex Leukemia PML/RARa, AML/ETO and BCR/ABL kits (Seegene, Seoul, Korea). DNA from the BM aspirates was isolated using the QIAamp DNA blood mini kit (Qiagen). FLT3 mutations were confirmed using a Seeplex FLT3 Genotyping kit (Seegene). These FLT3 mutations were classified as internal tandem duplication (ITD) mutations or mutation in the tyrosine kinase domain (TKD).
The sample was collected from each patient at diagnosis, and follow-up samples were collected in 6 patients with mutations. We analyzed 90 BM samples from 83 AML patients to detect N-ras mutations in codons 12, 13, and 61 using the pyrosequencing; all data were subsequently confirmed by direct sequencing. DNA from the BM aspirates was prepared using the QIAamp DNA blood mini kit (Qiagen). Mutations in the N-ras were detected by pyrosequencing using a TheraScreen NRAS Pyro kit (Qiagen). PCR templates for pyrosequencing were amplified from genomic DNA (10 ng) using the Pyro Mark PCR Master mix (Qiagen) and 5 pmol of each primer in a total reaction volume of 25 µL. Following PCR amplification, the biotinylated PCR products were placed in 24-well plates and bound to streptavidin-coated sepharose beads (GE Healthcare, Piscataway, NJ, USA). The PCR products were denatured, and the non-biotinylated fragments were washed from the beads using the Pyromark Q24 Vacuum Workstation (Qiagen). The beads were then resuspended in annealing buffer (24.2 µL) containing 0.4 pmol of the sequencing primer. Pyrosequencing was performed using the Pyro Gold Q24 reagents (Qiagen), using dispensations based on the target sequence with the Pyromark Q24 system. Raw data files were imported into Pyromark Q24 software (version 2.0; Qiagen) for further analysis following pyrosequencing.
The pyrosequencing data were confirmed by direct sequencing (Macrogen, Seoul, Korea). For the direct sequencing, 100 ng of genomic DNA from the patients was used for PCR with AccuPower PCR PreMix (Bioneer, Seoul, Korea). An initial preheating at 94℃ for 10 min was followed by denaturation at 94℃ for 40 sec, 58℃ for 40 sec, and 72℃ for 40 sec for 35 cycles and a final extension at 72℃ for 7 min. The primer sets used were forward primer 5'-GTGAGGCCGATATTAATCCG-3' and reverse primer 5'-ACAGTCACGCTACTATGGCC-3' for exon 1 to amplify codons 12 and 13 and forward primer 5'-GTTATAGATGGTGAAACCTG-3' and reverse primer 5'-GCTCTATCTTCCCTAGTGTG-3' for exon 2 to amplify codon 61. The PCR products were analyzed by direct sequencing using the exon 1 forward primer and the exon 2 reverse primer (Fig. 1).
The frequency of clinical characteristics was analyzed by Pearson's χ2 test. Differences in mean variables were analyzed by Mann-Whitney statistics. Cross tabulation was used in comparison between direct sequencing and pyrosequencing. All statistical analyses were performed using the SPSS software package version 17.0 (SPSS Inc., Chicago, IL, USA). For all the analyses, P values <0.05 were considered statistically significant.
The incidence of mutation in the N-ras gene was 7.2% in the AML patients. Mann-Whitney statistics revealed that the group of patients with N-ras mutations (6 patients) showed higher hemoglobin levels (P=0.022), and a higher prevalence rate of FLT3 mutations in patients with N-ras mutations was demonstrated by the Pearson's χ2 test (P=0.003). Among the 83 patients, 15 patients had FLT3 mutations (18.1%) and 13 of them showed ITD mutations. Two patients had TKD mutations. The incidence of FLT3 mutations in patients with wild-type N-ras was 16.9% (13 of 77 patients) and 33.3% (2 of 6 patients) in patients with the N-ras mutation (Table 1). Other factors were not statistically correlated with the presence of N-ras mutations (Table 1). All patients with mutations were diagnosed with de novo AML. Five of 6 patients were diagnosed with AML, not otherwise specified (AML, NOS) and 4 of these 6 patients had normal karyotypes (Tables 2, 3).
We observed 3 cases with mutated N-ras codon 12 (3.6%), 2 cases with mutated codon 13 (2.4%), and 1 case with mutated codon 61 (1.2%). None of the patients with mutations showed homozygous mutations. The base substitution in codon 12 subsequently led to an amino acid change from wild-type glycine to serine, cysteine, or aspartic acid (G12S, G12C, and G12D). Mutations at codon 13 also induced an amino acid substitution of wild-type glycine to aspartic acid or arginine (G13D and G13R). The mutation in codon 61 caused the glutamine to arginine substitution (Q61R).
We performed pyrosequencing and direct sequencing for all 83 patients. The BM study was performed at initial diagnosis, and the patients who received chemotherapy were re-evaluated for evidence of leukemia or CR after chemotherapy. Seven N-ras mutations were detected in 6 cases (7.2%) by both sequencing methods, and there was no difference in the detection rate (Fig. 2). The percentage of N-ras mutant DNA assayed for codon 12, 13, and 61 mutations by pyrosequencing was 23%-49% (Table 3). In 5 patients, the N-ras mutation disappeared from the BM after the induction chemotherapy, and morphological remission in the BM was also evident. Although there was no evidence of leukemic blasts in the BM, the N-ras mutation in codon 13 persisted after the induction therapy in 1 patient (sample no. 46). In this patient, the mutated allele burden was similar to that at initial diagnosis (initial, 39%; after induction chemotherapy, 33%) (Table 3), and the mutation disappeared after the first consolidation therapy. Of these 6 patients, 4 died, 1 was associated with treatment failure, and only 1 patient reached CR after BMT (Table 3).
Here, we studied the incidence of N-ras mutations in adult patients with AML. The incidence of N-ras mutation was 7.2% in the adult patients with AML examined at a single Korean institution. Recent studies have shown that N-ras mutation is present in AML patients in the range of 7.03-20.26%, and this value is reduced in Asian countries [6-13] (Table 4).
Compared to the total patient pool in this study, the patients with N-ras mutations (6 patients) showed higher hemoglobin levels (P=0.022) and a higher prevalence of FLT3 mutations (P=0.003). Other factors did not correlate with the presence of N-ras mutations.
We identified both N-ras mutation and FLT3-TKD mutation in 2 patients that presented with normal karyotypes. Whitman et al. [20] reported that younger adults with de novo CN-AML and FLT3-TKD mutations in D835/I836 are associated with worse outcome than those without FLT3-TKD mutations. A previous report by Illmer et al. [21] stated that AML patients with high Ras activity were highly sensitive to high doses of Ara-C and that Ras activity may overcome the poor prognosis in younger patients even in the presence of FLT3 mutations. In our study, case 71 had both N-ras mutation and FLT3-TKD mutation, and she was treated with palliative low doses of Ara-C because of her old age (81 yr old); she passed away 1 month after diagnosis. The other patient with both mutations (sample no. 58) who was younger (47 yr old) showed induction failure and demonstrated CR after a high dose of Ara-C treatment and allogenic BMT. These findings are consistent with the conjecture that aggressive treatments are required for the patients with N-ras and FLT3-TKD mutations.
In this study, all N-ras mutations were observed in de novo AML, but 94.0% of the enrolled patients were diagnosed with de novo AML. Therefore, the result could not reflect a relationship between the subtype of AML and the incidence of N-ras mutation. Two of the 6 patients were diagnosed with acute myelomonocytic/monocytic leukemia. Several studies have reported that N-ras mutations promote myeloid maturation defects with relative sparing of the monocyte lineage. In addition, Ras mutations are particularly prevalent in leukemia and preleukemia with monocytic characteristics [3, 5, 6, 22].
All point mutations in N-ras induced an amino acid change. In 3 patients, a G→A base substitution in codon 12 and 13 was detected; this is the most commonly reported base substitution in Ras mutations in AML [23, 24]. The glycine residue located in position 12 (Gly12) lies close to the "finger loop" of the GTPase activating proteins (GAP) that complements the active site of Ras. Any mutation at this position results in sterical interference with the geometry of the transition state, in which GTP is hydrolyzed in the presence of GAP fingers [1]. It has been reported that G12D shortens disease latency rather than contributing to tumorigenesis [25, 26]. We found that 2 of 3 patients with N-ras mutations in codon 12 died within 1 month after diagnosis; one of them had a G12D mutation. The other patient revealed re-induction failure and was lost to follow up (Table 3).
All N-ras mutations disappeared during chemotherapy, thereby suggesting a relationship between the presence of N-ras mutation and disease status. In one patient (case 46), leukemic blasts were not detected in BM, and chromosomal abnormalities present at initial diagnosis (46,XY,del(9)(q21q22)[25]/46,XY[5]) also disappeared after the induction chemotherapy. Nevertheless, the N-ras mutation persisted after the induction chemotherapy, and the level of the mutated gene (33%) was similar to the level at initial diagnosis (39%) (Table 3). After first consolidation chemotherapy, direct sequencing and pyrosequencing in case 46 did not detect the N-ras mutation. In the study by Bacher et al. [5], they concluded that N-ras mutations were not a good marker for follow-up control [5]. However, our study shows that monitoring N-ras mutations can be a more sensitive indicator of therapeutic response than morphologic or conventional cytogenetic studies.
In this study, pyrosequencing and direct sequencing showed same efficiencies with respect to their ability to detect mutations. During the therapeutic period, the number of leukemic cells decreases, and, in some cases, it is difficult to discriminate these cells from normal early precursor cells. A low limit of detection (LOD) of pyrosequencing is important in cases with rare mutations as well as in follow-up studies for early detection of relapse in patients with AML [17, 27]. Athanasios et al. [16] determined that the LOD for Sanger sequencing, pyrosequencing, and melting curve analysis is approximately 15-20%, 5%, and 10% mutant alleles, respectively. Another advantage of pyrosequencing is that it can read a starting sequence from the first nucleotide adjacent to a pyrosequencing primer without a noise signal. In addition, pyrosequencing is a simple, one-step method that is efficient because 24 samples can be analyzed in <40 min. Pyrosequencing is a simple method compared with Sanger sequencing and does not require a separate purification step or gel electrophoresis after PCR. However, there is a limitation in pyrosequencing kits because only a few positions (≤40-50 nucleotides) can be simultaneously analyzed, and it costs more than other methods (100,000-150,000 KRW/sample) [17, 28, 29].
In our study, the numbers of patients and N-ras mutation cases were too small to make any statistical conclusions. In addition, because the patients received different treatments, it was difficult to compare the outcome and prognostic effect of the N-ras mutations. Many factors may contribute to the clinical outcome associated with the presence of N-ras mutations, and our analysis investigated only a few of them.
This study is important because there are no recent reports analyzing the presence of N-ras mutations in the Korean patients with hematologic malignancies. This study identified the incidence of N-ras mutations (7.2%) in patients with AML at a single Korean institution and revealed the relationship between the presence of N-ras mutation and the therapeutic response. We also evaluated the pyrosequencing kit for N-ras mutation detection as a confirmatory test to identify specific mutations. The same efficiency with respect to the detection of N-ras mutations was determined for pyrosequencing and direct sequencing. Because pyrosequencing provides quantitative data and information regarding the mutation type, it is a useful tool for detecting malignant cells and for following patients' progress. Finally, it may be appropriate for large-scale applications in clinical settings for the analysis of hematologic malignancies.
References
1. Malumbres M, Barbacid M. RAS oncogenes: the first 30 years. Nat Rev Cancer. 2003; 3:459–465. PMID: 12778136.


2. Karnoub AE, Weinberg RA. Ras oncogenes: split personalities. Nat Rev Mol Cell Biol. 2008; 9:517–531. PMID: 18568040.


3. Neubauer A, Dodge RK, George SL, Davey FR, Silver RT, Schiffer CA, et al. Prognostic importance of mutations in the ras proto-oncogenes in de novo acute myeloid leukemia. Blood. 1994; 83:1603–1611. PMID: 8123851.


4. De Melo MB, Lorand-Metze I, Lima CS, Saad ST, Costa FF. N-ras gene point mutations in Brazilian acute myelogenous leukemia patients correlate with a poor prognosis. Leuk Lymphoma. 1997; 24:309–317. PMID: 9156660.
5. Bacher U, Haferlach T, Schoch C, Kern W, Schnittger S. Implications of NRAS mutations in AML: a study of 2502 patients. Blood. 2006; 107:3847–3853. PMID: 16434492.


6. Bowen DT, Frew ME, Hills R, Gale RE, Wheatley K, Groves MJ, et al. RAS mutation in acute myeloid leukemia is associated with distinct cytogenetic subgroups but does not influence outcome in patients younger than 60 years. Blood. 2005; 106:2113–2119. PMID: 15951308.


7. Ritter M, Kim TD, Lisske P, Thiede C, Schaich M, Neubauer A. Prognostic significance of N-RAS and K-RAS mutations in 232 patients with acute myeloid leukemia. Haematologica. 2004; 89:1397–1399. PMID: 15531466.
8. Stirewalt DL, Kopecky KJ, Meshinchi S, Appelbaum FR, Slovak ML, Willman CL, et al. FLT3, RAS, and TP53 mutations in elderly patients with acute myeloid leukemia. Blood. 2001; 97:3589–3595. PMID: 11369655.


9. Schlenk RF, Döhner K, Krauter J, Fröhling S, Corbacioglu A, Bullinger L, et al. Mutations and treatment outcome in cytogenetically normal acute myeloid leukemia. N Engl J Med. 2008; 358:1909–1918. PMID: 18450602.


10. Ishikawa Y, Kiyoi H, Tsujimura A, Miyawaki S, Miyazaki Y, Kuriyama K, et al. Comprehensive analysis of cooperative gene mutations between class I and class II in de novo acute myeloid leukemia. Eur J Haematol. 2009; 83:90–98. PMID: 19309322.
11. Patel JP, Gönen M, Figueroa ME, Fernandez H, Sun Z, Racevskis J, et al. Prognostic relevance of integrated genetic profiling in acute myeloid leukemia. N Engl J Med. 2012; 366:1079–1089. PMID: 22417203.


12. Rockova V, Abbas S, Wouters BJ, Erpelinck CA, Beverloo HB, Delwel R, et al. Risk stratification of intermediate-risk acute myeloid leukemia: integrative analysis of a multitude of gene mutation and gene expression markers. Blood. 2011; 118:1069–1076. PMID: 21596848.


13. Shen Y, Zhu YM, Fan X, Shi JY, Wang QR, Yan XJ, et al. Gene mutation patterns and their prognostic impact in a cohort of 1185 patients with acute myeloid leukemia. Blood. 2011; 118:5593–5603. PMID: 21881046.


14. Miyauchi J, Asada M, Sasaki M, Tsunematsu Y, Kojima S, Mizutani S, et al. Mutations of the N-ras gene in juvenile chronic myelogenous leukemia. Blood. 1994; 83:2248–2254. PMID: 8161790.


15. Ahmadian A, Ehn M, Hober S. Pyrosequencing: history, biochemistry and future. Clin Chim Acta. 2006; 363:83–94. PMID: 16165119.


16. Tsiatis AC, Norris-Kirby A, Rich RG, Hafez MJ, Gocke CD, Eshleman JR, et al. Comparison of Sanger sequencing, pyrosequencing, and melting curve analysis for the detection of KRAS mutations: diagnostic and clinical implications. J Mol Diagn. 2010; 12:425–432. PMID: 20431034.
17. Ogino S, Kawasaki T, Brahmandam M, Yan L, Cantor M, Namgyal C, et al. Sensitive sequencing method for KRAS mutation detection by Pyrosequencing. J Mol Diagn. 2005; 7:413–421. PMID: 16049314.


18. Swerdlow SH, Campo E, Harris NL, Jaffe ES, Pileri SA, Stein H, editors. WHO classification of tumours of haematopoietic and lymphoid tissues. 2008. 4th ed. Lyon: IARC;p. 110–155.
19. Shaffer LG, Slovak ML, Campbell LJ, editors. ISCN 2009: An international system for cytogenetic nomenclature (2009). 2009. Basel: S Karger.
20. Whitman SP, Ruppert AS, Radmacher MD, Mrózek K, Paschka P, Langer C, et al. FLT3 D835/I836 mutations are associated with poor disease-free survival and a distinct gene-expression signature among younger adults with de novo cytogenetically normal acute myeloid leukemia lacking FLT3 internal tandem duplications. Blood. 2008; 111:1552–1559. PMID: 17940205.


21. Illmer T, Thiede C, Fredersdorf A, Stadler S, Neubauer A, Ehninger G, et al. Activation of the RAS pathway is predictive for a chemosensitive phenotype of acute myelogenous leukemia blasts. Clin Cancer Res. 2005; 11:3217–3224. PMID: 15867216.


22. Darley RL, Burnett AK. Mutant RAS inhibits neutrophil but not macrophage differentiation and allows continued growth of neutrophil precursors. Exp Hematol. 1999; 27:1599–1608. PMID: 10560907.


23. Speletas M, Arvanitidi K, Tzoanopoulos D, Tsironidou V, Pardali E, Aggeli C, et al. Rapid mutational analysis of N-ras proto-oncogene in hematologic malignancies: study of 77 Greek patients. Haematologica. 2001; 86:918–927. PMID: 11532619.
24. Auewarakul CU, Lauhakirti D, Tocharoentanaphol C. Frequency of RAS gene mutation and its cooperative genetic events in Southeast Asian adult acute myeloid leukemia. Eur J Haematol. 2006; 77:51–56. PMID: 16573741.


25. Tyner JW, Erickson H, Deininger MW, Willis SG, Eide CA, Levine RL, et al. High-throughput sequencing screen reveals novel, transforming RAS mutations in myeloid leukemia patients. Blood. 2009; 113:1749–1755. PMID: 19075190.


26. Chou FS, Wunderlich M, Griesinger A, Mulloy JC. N-Ras(G12D) induces features of stepwise transformation in preleukemic human umbilical cord blood cultures expressing the AML1-ETO fusion gene. Blood. 2011; 117:2237–2240. PMID: 21200020.


27. Sundström M, Edlund K, Lindell M, Glimelius B, Birgisson H, Micke P, et al. KRAS analysis in colorectal carcinoma: analytical aspects of Pyrosequencing and allele-specific PCR in clinical practice. BMC Cancer. 2010; 10:660. PMID: 21122130.


28. Voelkerding KV, Dames SA, Durtschi JD. Next-generation sequencing: from basic research to diagnostics. Clin Chem. 2009; 55:641–658. PMID: 19246620.


29. Sivertsson A, Platz A, Hansson J, Lundeberg J. Pyrosequencing as an alternative to single-strand conformation polymorphism analysis for detection of N-ras mutations in human melanoma metastases. Clin Chem. 2002; 48:2164–2170. PMID: 12446472.


Fig. 1
Schematic representation of the location of the direct sequencing primers on the N-ras codons 12, 13, and 61. Codons 12 and 13 are located in exon 1, and codon 61 is in exon 2. For the detection of mutations in codon 12 and 13, PCR products of 607 nucleotides were analyzed. Mutations in codon 61 were detected by sequencing the PCR product of exon 2 (248 nucleotides).
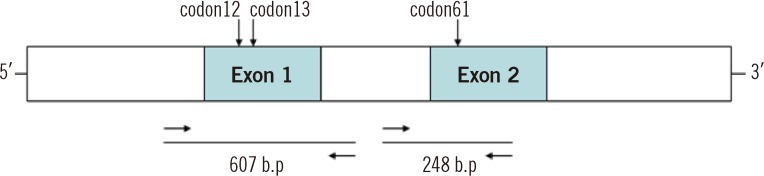
Fig. 2
N-ras mutations established by pyrosequencing and confirmed by direct sequencing. (A) Pyrogram showing the presence of mutation and quantitative data of mutated clones. The horizontal axis refers to the base, and the vertical axis represents the intensity of the fluorescent signal. The shaded regions represent the analyzed codon. The first box shows the analytic results of substitutions in the first base of the N-ras codons 12 and 13. The second box presents mutations in the second base of the N-ras codons 12 and 13. The third box shows a positive result for a mutation in the second base of codon 61. (B) All patient samples were confirmed by direct sequencing, and the plots showed the same results as pyrosequencing.
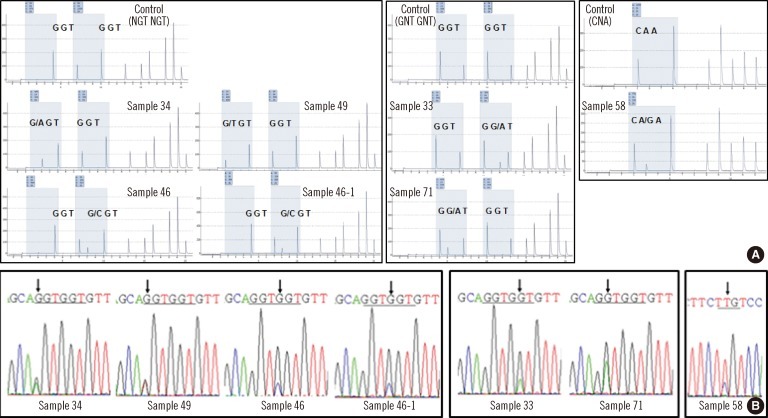
Table 3
Characteristics of AML patients with N-ras mutation and sequencing results
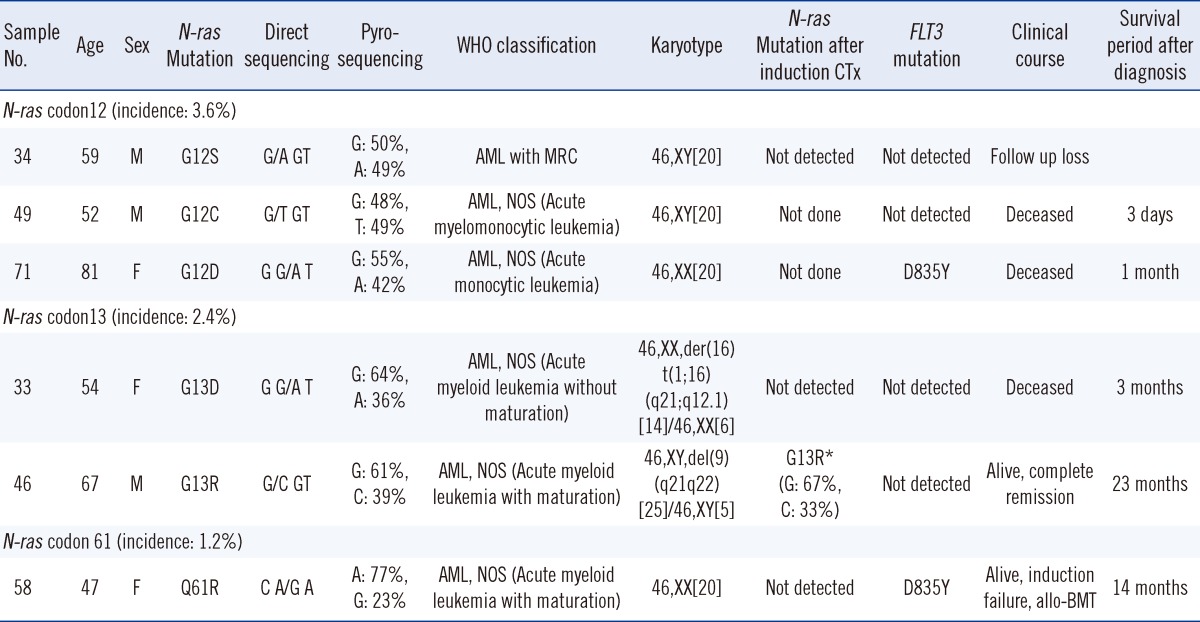
*After induction chemotherapy, the N-ras mutation in codon 13 persisted and the mutated allele burden was similar to that at initial diagnosis.
AML, NOS, AML, not otherwise specified.
Abbreviations: CTx, chemotherapy; BMT, bone marrow transplantation; AML with MRC, AML with myelodysplasia-related changes; D835Y, FLT3 kinase domain point mutation.