Abstract
Background
Recombinant human bone morphogenetic proteins (rhBMPs) have been widely used in regenerative therapies to promote bone formation. The production of rhBMPs using bacterial systems such as Escherichia coli (E. coli) is estimated to facilitate clinical applications by lowering the cost without compromising biological activity. In clinical practice, rhBMP-2 and osteoconductive carriers (e.g., hydroxyapatite [HA] and bovine bone xenograft) are used together. This study examined the effect of E. coli-derived rhBMP-2 combined with porous HA-based ceramics on calvarial defect in rabbits.
Methods
Six adult male New Zealand white rabbits were used in this study. The experimental groups were divided into the following 4 groups: untreated (NC), bovine bone graft (BO), porous HA (HA) and porous HA with rhBMP-2 (HA-BMP). Four transosseous defects of 8 mm in diameter were prepared using stainless steel trephine bur in the frontal and parietal bones. Histological and histomorphometric analyses at 4 weeks after surgery revealed significant new bone formation by porous HA alone.
Results
HA-BMP showed significantly higher degree of bone formation compared with BO and HA group (P<0.05). The average new bone formation % (new bone area per total defect area) of NC, BO, HA, and HA-BMP at 4-week after surgery were 12.65±5.89%, 29.63±6.99%, 28.86±6.17% and 49.56±8.23%, respectively. However, there was no statistical difference in the bone formation between HA and BO groups.
Bone morphogenetic proteins (BMPs) were first discovered in 1965, when Urist [1] observed the formation of ectopic bones by implanting decalcified bone matrix preparations subcutaneously or intramuscularly in rodent models. BMPs are consisted of more than 20 proteins of multi-functional growth factors that belong to transforming growth factor-beta (TGF-β) superfamily. BMPs are known to induce bone formation analogous to endochondral ossification by acting on undifferentiated mesenchymal cells in vivo.[23] Among these family proteins, BMP-2, 4, and 7 have been demonstrated to play important roles in osteogenic process.[4] Recombinant human BMPs (rhBMPs) are often utilized in regenerative medicine to enhance reconstruction of functional bones. Among numerous reports using animal models that revealed the promotion of cranial, mandibular, and iliac bone regeneration by rhBMP-2, Sigurdsson et al.[5] identified the increased bone regeneration when rhBMP-2 was applied on periodontal defects in beagle dogs, suggesting the usefulness of rhBMP-2 in periodontal regenerative therapies.
For the clinical effectiveness, therapeutic BMP proteins are required to not only possess sufficient osteogenic capacity but also need to be easily mass-producible for the economic efficiency. However the yield of purified BMPs from fresh bones are about 0.1 µg/kg of bone.[6] Human BMP-2 was initially cloned in the late 1980's and the recombinant protein is now being produced using in vitro culture systems of animal cells, mostly Chinese hamster ovary (CHO) cells, or prokaryotic cells including Escherichia coli (E. coli). Although the rhBMPs derived from CHO cells are effective in promoting bone formation, high cost for the production prevents these rhBMPs from the routine clinical applications.[7] The E. coli-derived rhBMP-2 can be mass-produced by renaturing the protein following purification from inclusion bodies, which makes this rhBMP-2 a potential solution for the cost problem.[8] Although E. coli-derived BMP-2 is not glycosylated as that purified from animal cells, its biological activities are not significantly different.[910]
For the clinical application of rhBMP-2, the protein should be combined with carrier material for optimal biocompatibility, space maintenance, as well as tissue integration to maximize osteoconductivity.[5] Previous studies tested several carrier materials such as absorbable collagen sponge (ACS), hydroxyapatite (HA), and beta-tricalcium phosphate (β-TCP). Although ACS is an U.S. Food and Drug Administration-approved carrier, large amount of rhBMP-2 is required for clinical use due to its poor affinity to rhBMP-2. [11] The β-TCP is highly osteoconductive, biocompatible, and completely resorbable.[12] HA is well-known osteoconductive and biocompatible material with low absorption rate.[13] Since each material has respective merits and limitations, developing optimal carrier is a challenge for the regeneration of bone.
The osteoconductivity of HA is considerably affected by the microstructure of the material. For example, porous structures allow ingrowth of cells and microvessels that induce bone formation, and the optimal size of pore for enhancing bone formation has been estimated between 150 and 500 µm.[1415] The HA scaffolds are often combined with supportive osteoinductive molecules such as BMPs. [16] In the present study, we assessed fundamental osteoconductivity of porous HA combined with E. coli-derived BMP-2 using a rabbit calvarial defect model. Our results imply potential clinical usefulness of porous HA loaded with E. coli-derived BMP-2.
In this experiment, porous HA (Bongros; Daewoong Pharmaceutical Co. Ltd., Seongnam, Korea) and bovine bone xenograft (Bio-Oss; Geistlich, Wolhusen, Switzerland) were used. Bovine bone xenograft was obtained from Geistlich (Bio-Oss; Geistlich). Recombinant human BMP2 was obtained from Daewoong Pharmaceutical Co. Ltd. Expression and purification of rhBMP-2 from E. coli cDNA encoding the mature form of the BMP-2 protein was amplified via polymerase chain reaction and subcloned into an E. coli expression vector regulated by the T7 promoter. The rhBMP-2 was loaded to porous HA with immersion method. Microscopic surface structures of porous HA and bovine bone xenograft were analyzed with fscanning electron microscope (SU8220; Hitachi High Technologies, Tokyo, Japan) following platinum-coating of the samples. The qualitative as well as quantitative characteristics of graft material compositions were analyzed by energy dispersive X-ray spectrometer (X-ManN50 011; HORIBA Ltd., Kyoto, Japan).
Six adult male New Zealand white rabbits weighing 2.6 to 3.0 kg were obtained from Central Laboratory Animals (Seoul, Korea) and were adapted to the animal facility environment for 2 weeks. For surgery, general anesthesia was induced by intramuscular injection of zolazepam (0.2 mL/kg; Zoletil, Virbac Korea, Seoul, Korea) and xylazine (0.25 mL/kg; Rompun, Bayer Korea Co., Seoul, Korea). After shaving, a complementary infiltrative anesthesia of the surgical sites was achieved using 2% lidocaine with 1/100,000 epinephrine (Yuhan, Seoul, Korea) to control bleeding. After the introduction of sagittal incision through the skin and periosteum at the midline of the calvaria, 4 standardized transosseous defects of 8 mm in diameter were prepared using stainless steel trephine bur in the frontal and parietal bones under profuse irrigation with sterile saline solution.
Defects were filled with following graft materials; 25 mg of porous HA soaked in 50 µL saline (HA group), 25 mg of HA soaked with 70 µg of E. coli-derived rh-BMP-2 dissolved in 50 µL saline (HA-BMP group), and 25 mg of bovine bone xenograft soaked in 50 µL saline (BO group). The unfilled defect served as negative control (NC group). The surgery for the formation and filling of calvarial defects were depicted in Figure 1. Surgical sites were covered with absorbable collagen membrane (CollaTape; Zimmer, Carlsbad, CA, California) and were sutured using braided polyglycolic acid sutures (5-0 surgifit; AILEE Co., Busan, Korea) and monofilament sutures (4-0 nylon Blue; AILEE Co.). Antibiotics (Baytril, Bayer Korea Co.) and analgesics (Nobin, Bayer Korea Co.) were injected intramuscularly for 3 days to prevent postsurgical infection and to control pain. The animal experimental protocols were approved by the Institutional Animal Care and Use Committee at Kyungpook National University.
At 4 weeks after surgery, animals were sacrificed and calvariae were removed. The tissue samples were fixed in 4% neutral-buffered paraformaldehyde, decalcified in 10% ethylenediaminetetraacetic acid (EDTA) for 1 month, and embedded in paraffin. The 3-µm-thick sections were prepared using a Leica RM2245 microtome (Leica Microsystems, Bannockbrun, IL, USA). After hematoxylin and eosin (H & E) staining, histological evaluation was performed upon observation under Olympus BX53 microscope (Olympus, Tokyo, Japan) equipped with 10×0.30 UPLFLN objective lens (Olympus). Images were captured using a digital camera (Olympus) attached to the microscope. Histomorphometric analyses were performed using the I-solution image analysis software (iMTechnology, Daejeon, Korea) to quantitatively calculate the area of new bone formation in the defects.
Statistical analyses were performed using the PASW 22 package (SPSS Inc., Chicago, IL, USA). A nonparametric Kruskal-Wallis analysis of variance (ANOVA) test was performed to evaluate differences in new bone formation area among the groups. The significance of any differences in bone regeneration between the groups was evaluated by the Mann-Whitney U-test. Results with P-values less than 0.05 were considered statistically significant.
The surface properties of bone graft materials used in this study were observed by scanning electron microscopy (SEM) at ×100 magnification (Fig. 2). The examination of surface morphology revealed pores of 300 to 500 µm in diameter for porous HA, which was absent in bovine bone xenograft. Energy dispersive X-ray spectrometry (EDS) analyses on the composition of graft materials showed that calcium to phosphate ratio (Ca/P) of porous HA and bovine bone xenograft was 2.934 and 2.223, respectively (Table 1). Carbon, oxygen, calcium, and phosphate composed the majority of both bone graft materials, with small amount of sodium and magnesium detected in bovine bone xenograft.
After surgery for the defect formation and filling with graft materials combined with the E. coli-derived rhBMP-2, rabbits (n=6) showed no postoperative complications such as wound dehiscence, edema and bleeding. At 4 weeks after surgery, calvarial samples were prepared for histological analyses. The H & E staining revealed the formation of new bones in all experimental groups, but none of them exhibited complete bony bridging (Fig. 3A). New bone formation was more evident in the regions near the defect margins, although it was also observed in central areas of the defects in some samples (Fig. 3B). All groups with bone graft materials showed dramatic increase in the mineralized area compared with unfilled control. Importantly, HA-BMP group showed significantly higher degree of bone formation compared with HA group and BO group, suggesting the efficacy of promoting bone formation was remarkably enhanced by loading porous HA particles with E. coli-derived rhBMP-2. The average new bone formation % (new bone area per total defect area) of NC, BO, HA, and HA-BMP group at 4-week after surgery were 12.65±5.89%, 29.63±6.99%, 28.86±6.17%, and 49.56±8.23%, respectively (Fig. 4). The effect of E. coli-derived rhBMP-2 on bone regeneration was further confirmed by the micro computed tomography analysis (Fig. 5). The inclusion of bone graft materials induced statistically significant increase in the bone formation. However, there was no statistical difference in the bone formation induced by porous HA (HA group) and bovine bone xenograft (BO group). The new bone formation % for HA-BMP group was significantly higher than HA group (Kruskal-Wallis ANOVA, P=0.005).
Since adipogenesis would hamper bone quality during bone regeneration, the formation of adipocytes were scrutinized from the H & E-stained tissue sections (Fig. 6). Among the six rabbits implanted with porous HA and E. coli-derived rhBMP-2, adipogenesis was observed in one rabbit. Since adipogenesis was not discovered in any of control rabbit or those treated with HA or bone xenograft alone, it is believed that adipogenesis was originated due to the presence of rhBMP-2.
In the present study, rabbit calvarial defect model was utilized to assess the effect of porous HA combined with E. coli-derived rhBMP-2 on bone regeneration. When observed at 4 weeks after surgery, porous HA loaded with E. coli-derived rhBMP-2 was more effective than using HA alone or conventional deproteinized bovine bone xenograft. Until present, surface structures of bone graft material in micro- or nano-scale have been considered important for the biocompatibility of material. Optimal porosity and pore size can provide osteoblasts with favorable conditions for adhesion and osteogenesis. The synthetic porous HA used in the current study possessed pores of 300 µm in diameter, with 80% porosity and 3-dimensionally (3D) interconnected porous structure. The 3D structures of the scaffold that mimics those of extracellular matrices provide cells with essential supports for its attachment, growth and differentiation, while the porosity allows immigration of cells and nutrient flow.[1718] Liebschner[19] suggested that the pore size of a scaffold needs to be greater than 30 µm to allow bone ingrowth. Others reported that significant bone ingrowth could occur when the size of pores was at least 100 µm.[2021] Furthermore, it was suggested that pores exceeding 300 µm in size induce osteogenesis with capillary formation, while smaller pores underwent osteochondral ossification.[22] Combined, the porous HA used in the present study provided optimal osteoconductive environment that served as carrier for rhBMP-2. Nevertheless, additional studies for the effect of various pore sizes in porous HA would be needed, since the bovine bone xenograft with no porous structures exhibited similar bone-forming effect compared with porous HA. Clinically, both of the two graft materials are estimated to be appropriate scaffolds, holding spaces for ingrowth of host bone marrow.
Although porous HA was loaded with rhBMP-2 by immersion in saline containing the protein in the current study, other methods have been suggested with different loading efficiency and release profile.[23] The immersion method is currently most widely used because of the ease of the procedure as well as high BMP loading efficiency. However, incorporation of BMP during HA precipitation exhibited prolonged BMP release profile that is clinically desirable, although it was not as effective in BMP loading as the immersion method.[23] Thus, in addition to the variations in HA carrier characteristics, modification of BMP loading methods might be required in future studies to achieve optimal BMP-carrier combination for clinical use.
Earlier report suggested that rhBMP-2 derived from the Chinese hamster ovarian cells increases not only osteogenic differentiation but also adipogenic potential in a dose-dependent manner.[24] The increased adipogenesis might be detrimental, since the bone quality would be compromised by the high concentration of rhBMP-2. Similarly, Park et al.[25] reported increased adipogenesis in vitro, when human alveolar bone-derived stromal cells were treated with high concentrations of E. coli-derived rhBMP-2. In the present experiments, adipogenesis was observed in one out of six rabbits treated with E. coli-derived rhBMP-2 (Fig. 6). Further studies on porous HA loaded with various concentrations of rhBMP-2 would clarify the possible correlation between adipogenesis and the use of E. coli-derived rhBMP-2.
Yoshida et al.[26] previously suggested that the combination of porous HA and animal cell-derived rhBMP-2 was more effective than the single use of HA for recovery of rabbit mandibular defect. In the present study, we showed that the addition of E. coli-derived rhBMP-2 significantly promoted the osteoconductivity of HA. Thus, the use of E. coli-derived rhBMP-2 that is more cost-effective without compromising the osteogenic potential of the animal cell-derived rhBMP-2 might be a desirable substitute for the regenerative therapies especially when a large bony defect exists.
Figures and Tables
Fig. 1
Clinical photograph of the surgery. (A) Sagittal incisions were made along the midline of the calvaria in six rabbits under anesthesia. Four transosseous defects of 8 mm in diameter were prepared using stainless steel trephine bur in the frontal and parietal bones. (B) Defects were filled with porous hydroxyapatite (HA), porous HA with recombinant human bone morphogenetic protein-2, bovine bone xenograft, or left untreated for control.
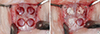
Fig. 2
Surface structures of porous hydroxyapatite (HA) and bovine bone xenograft (BO). Graft materials were coated with platinum and were subjected to the scanning electron microscopy. Microscopic structures of porous HA (A) and bovine bone xenograft (B) were shown in ×100 magnification. Scale bars indicate 500 µm.
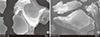
Fig. 3
Histological evaluation of bone regeneration. Rabbit calvarial defects were either untreated (negative control [NC]), filled with bovine bone xenograft (BO; positive control), filled with porous hydroxyapatite (HA), or filled with porous HA plus Escherichia coli-derived recombinant human bone morphogenetic protein-2 (rhBMP-2). At 4 weeks after surgery, calvariae were removed, fixed, decalcified, and embedded in paraffin. Tissue sections of 3 m thickness were prepared using a microtome. (A) The tissue sections were subjected to hematoxylin and eosin staining. Microscopic images with along the coronal plane of the calvarial defects were obtained (×12.5 magnification). (B) Higher magnification (×40) images of the boxed areas in (A) area shown. Scale bars indicate 500 µm. Representative images from 6 rabbits are presented.
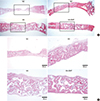
Fig. 4
Histomorphometric evaluation of bone regeneration. Quantitative analysis of new bone formation was performed by calculating new bone area per total defect area using the I-solution software (IMT i-Solution Inc., Vancouver, BC, Canada) as described in materials and methods. Data are mean±standard error of the mean. *P<0.05 vs. untreated control. **P<0.05 vs. porous hydroxyapatite (HA) alone or bovine bone xenograft. rhBMP-2, recombinant human bone morphogenetic protein-2.
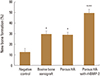
Fig. 5
The effect of Escherichia coli-derived recombinant human bone morphogenetic protein-2 in bone regeneration in vivo. Calvariae were subjected to micro-computer tomography analysis. Representative data from per group are shown (newly formed bone; pink: hydroxyapatite (HA) group, blue: HA-bone morphogenetic protein group, green: bovine bone xenograft group, and yellow: negative control group). Although the HA particles are not fully resorbed after 4 weeks after surgery, but are discernible from newly formed bones in defect area.
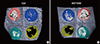
Fig. 6
Histologic analysis for the adipogenesis induced by Escherichia coli-derived recombinant human bone morphogenetic protein-2 (rhBMP-2) in vivo. Among the 6 samples per group, adipogenesis was only observed in 1 slide in porous hydroxyapatite plus rhBMP-2 group (hematoxylin and eosin [H & E], ×100 magnification). Scale bar indicates 500 µm. The arrowhead indicates adipocytes.
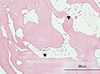
ACKNOWLEDGEMENT
This study was supported by research grants from CGBio. The funder had no role in study design, data collection and analysis, the decision to publish, or the preparation of the manuscript. This work was supported by the National Research Foundation of Korea (NRF) grant funded by the Korea government (MSIP, 2008-0062282).
References
2. Reddi AH, Cunningham NS. Initiation and promotion of bone differentiation by bone morphogenetic proteins. J Bone Miner Res. 1993; 8:Suppl 2. S499–S502.


3. Inoda H, Yamamoto G, Hattori T. Histological investigation of osteoinductive properties of rh-BMP2 in a rat calvarial bone defect model. J Craniomaxillofac Surg. 2004; 32:365–369.


4. Hogan BL. Bone morphogenetic proteins: multifunctional regulators of vertebrate development. Genes Dev. 1996; 10:1580–1594.


5. Sigurdsson TJ, Nygaard L, Tatakis DN, et al. Periodontal repair in dogs: evaluation of rhBMP-2 carriers. Int J Periodontics Restorative Dent. 1996; 16:524–537.
6. Urist MR, Huo YK, Brownell AG, et al. Purification of bovine bone morphogenetic protein by hydroxyapatite chromatography. Proc Natl Acad Sci U S A. 1984; 81:371–375.


7. Israel DI, Nove J, Kerns KM, et al. Expression and characterization of bone morphogenetic protein-2 in Chinese hamster ovary cells. Growth Factors. 1992; 7:139–150.


8. Lee JH, Jang SJ, Koo TY, et al. Expression, purification and osteogenic bioactivity of recombinant human BMP-2 derived by Escherichia coli. Tissue Eng Regen Med. 2011; 8:8–15.
9. Vallejo LF, Brokelmann M, Marten S, et al. Renaturation and purification of bone morphogenetic protein-2 produced as inclusion bodies in high-cell-density cultures of recombinant Escherichia coli. J Biotechnol. 2002; 94:185–194.


10. Lee J, Lee EN, Yoon J, et al. Comparative study of Chinese hamster ovary cell versus Escherichia coli-derived bone morphogenetic protein-2 using the critical-size supraalveolar peri-implant defect model. J Periodontol. 2013; 84:415–422.


11. Geiger M, Li RH, Friess W. Collagen sponges for bone regeneration with rhBMP-2. Adv Drug Deliv Rev. 2003; 55:1613–1629.


12. Daculsi G, LeGeros RZ, Nery E, et al. Transformation of biphasic calcium phosphate ceramics in vivo: ultrastructural and physicochemical characterization. J Biomed Mater Res. 1989; 23:883–894.


13. Lee JH, Hwang CJ, Song BW, et al. A prospective consecutive study of instrumented posterolateral lumbar fusion using synthetic hydroxyapatite (Bongros-HA) as a bone graft extender. J Biomed Mater Res A. 2009; 90:804–810.


14. Bignon A, Chouteau J, Chevalier J, et al. Effect of micro- and macroporosity of bone substitutes on their mechanical properties and cellular response. J Mater Sci Mater Med. 2003; 14:1089–1097.


15. Tsuruga E, Takita H, Itoh H, et al. Pore size of porous hydroxyapatite as the cell-substratum controls BMP-induced osteogenesis. J Biochem. 1997; 121:317–324.


16. Ripamonti U, Ma SS, van den Heever B, et al. Osteogenin, a bone morphogenetic protein, adsorbed on porous hydroxyapatite substrata, induces rapid bone differentiation in calvarial defects of adult primates. Plast Reconstr Surg. 1992; 90:382–393.


18. Murphy CM, Haugh MG, O'Brien FJ. The effect of mean pore size on cell attachment, proliferation and migration in collagen-glycosaminoglycan scaffolds for bone tissue engineering. Biomaterials. 2010; 31:461–466.


19. Liebschner MA. Biomechanical considerations of animal models used in tissue engineering of bone. Biomaterials. 2004; 25:1697–1714.


20. Jones JR, Hench LL. Factors affecting the structure and properties of bioactive foam scaffolds for tissue engineering. J Biomed Mater Res B Appl Biomater. 2004; 68:36–44.


21. Vallet-Regí M. Current trends on porous inorganic materials for biomedical applications. Chem Eng J. 2008; 137:1–3.


22. Karageorgiou V, Kaplan D. Porosity of 3D biomaterial scaffolds and osteogenesis. Biomaterials. 2005; 26:5474–5491.


23. Rohanizadeh R, Chung K. Hydroxyapatite as a carrier for bone morphogenetic protein. J Oral Implantol. 2011; 37:659–672.


24. Sciadini MF, Johnson KD. Evaluation of recombinant human bone morphogenetic protein-2 as a bone-graft substitute in a canine segmental defect model. J Orthop Res. 2000; 18:289–302.

