Abstract
Purpose
To identify potential differences in resting-state networks according to laterality of tinnitus using resting-state functional MRI (fMRI).
Materials and Methods
A total of 83 age-matched subjects consisting of 19 patients with right-sided tinnitus (Rt-T), 22 patients with left-sided tinnitus (Lt-T), 22 patients with bilateral tinnitus (Bil-T), and 20 healthy controls underwent resting-state blood oxygenation-level dependent fMRI scans. Independent component analysis was used to obtain the functional connectivities in the auditory network (AN) and the default mode network (DMN), which were compared between each group using the voxel-wise one-way ANOVA. In addition, lateralization of the auditory cortex was assessed within each group using a region of interest (ROI).
Results
Comparisons between tinnitus groups showed unusual clusters with different functional connectivities in the AN and the DMN. The Rt-T group had large clusters with higher functional connectivity in the right middle temporal gyrus and temporopolar area compared with the Lt-/Bil-T and control groups. ROI analysis showed that the Rt-/Lt-T groups had dominant functional connectivity in the right auditory cortex and the Bil-T and control groups had left-dominant auditory connectivity.
Tinnitus is defined as an auditory phantom perception without a corresponding external sound source. Tinnitus is one of the most common otologic symptoms, affecting 10–15% of people (123). One potential mechanism of tinnitus is the alteration of perception in neural auditory pathways. Therefore, tinnitus is often the focus of neuroscience studies involving functional MRI (fMRI). In the last decade, fMRI in tinnitus has focused on task-based neuronal stimulations using auditory and somatic modulation. One limitation of task-based fMRI in tinnitus is the bias from modulation processing. Because auditory or somatic modulation in a tinnitus patient is often too impaired to correctly perform the requested task, task-based fMRI studies in tinnitus patients are difficult to generalize.
Contrary to task-based fMRI, resting-state fMRI (rs-fMRI) is a relatively novel technique that has the potential to overcome many of these limitations. Because tinnitus involves consistently abnormal intrinsic neural activation, it is a suitable subject for rs-fMRI. Several previous studies demonstrated alterations of resting-state functional connectivity (FC) in tinnitus using magnetoencephalography (45). These studies reported different activities and connectivities in the auditory cortex networks of the tinnitus groups compared to the control groups. Additional research has explored altered connectivity in tinnitus using rs-fMRI (678910111213). Papers describing rs-fMRI use in patients with tinnitus have only recently started to get published. The primary findings of these studies were greater connectivity between the auditory network (AN) and the limbic area, alterations in the AN, and increased connectivity between the attention and auditory processing brain regions. However, existing studies are limited by heterogeneity and a small sample size. One of the roles of rs-fMRI in research is to demonstrate the variance of neuronal networks according to the clinical phenotype in neuropsychological disorders. Subjective tinnitus can be classified by the subgroup according to lateralization of tinnitus. Because tinnitus is considered a central nervous system problem, we anticipate that different clinical manifestations may be associated with different neuronal activities (141516). Previous studies using task-based fMRI and positron emission tomography (PET) supported the hypothesis that different neural activities are related to lateralization of tinnitus (1617181920). We hypothesized that neuronal connectivity will differ according to lateralization of tinnitus, and that rs-fMRI would be an effective tool for revealing these differences. Demonstration of neuronal connectivity according to lateralization will be helpful for objective measurement of lateralized tinnitus and implementation of effective tinnitus management strategies. Localization of neural activity in tinnitus is used as a target for applying localized treatments such as electrode implants or transc-ranial magnetic stimulation for the management of patients with intractable tinnitus. Therefore, the objective of this study is to evaluate lateralization of the AN in tinnitus patients, and furthermore, to identify potential differences in resting-state networks according to laterality of tinnitus.
We declare that all human and animal studies have been approved by the Institutional Review Board of the Kyung Hee University Hospital at Gangdong, and they have therefore been performed in accordance with the ethical standards laid down in the 1964 Declaration of Helsinki and its later amendments. All subjects provided informed consent to understand the purpose of the study and to undergo MR imaging.
This retrospective study included patients who were diagnosed with tinnitus at the tinnitus clinic in our institutes from 2011 to 2012. Inclusion criteria were as follows: 1) age under 65 years,2) continuous and chronic tinnitus for more than six months, and 3) moderate Tinnitus Handicap Inventory (THI) score higher than 38. Exclusion criteria were as follows: 1) diagnosis of a neuropsychological disorder including DSM-IV, 2) alcohol or drug addiction, 3) gross cochlear or retrocochlear abnormality on MRI, 4) hyperacusis, 5) presence of a cardiac pacemaker, claustrophobia, cochlear implant or any other issue that would contraindicate an MRI, and 6) presence of a parenchymal lesion that causes symptoms such as acoustic schwannoma, brain tumor or other parenchymal lesion. A total of 63 patients were enrolled in this study.
Healthy adults who were matched with the patient group by age and sex were recruited into the control group. Volunteers with normal findings based on pure tone audiometry were included. Finally, 19 patients with right-sided tinnitus (Rt-T) (mean age 51.3 years, 6 males), 22 patients with left-sided tinnitus (Lt-T; 51.3 years, 6 males), 22 patients with bilateral tinnitus (Bil-T; 54.1 years, 11 males) and 20 healthy control (51.0 years, 11 males) patients were enrolled in this study.
We assessed the clinical and otologic information including basic demographics such as age and sex, presence of hypertension, THI, Beck Depression Inventory (BDI), tinnitus pitch matching (Hz), duration of tinnitus, tinnitus type (pure tone or narrow band noise), and laterality (right-side, left-side, or bilateral).
rs-fMRI was acquired for all enrolled subjects using a 3.0 Tesla MRI system (Achieva, Philips Healthcare, Best, the Netherlands). To minimize unintended excitation during imaging, subjects were instructed to keep their eyes closed, relax their minds, and keep their heads still. Perception of MRI scanner noise was reduced with earplugs and headphones. Resting-state BOLD fMRI was acquired with a single-shot gradient-echo echo-planar imaging sequence using an 8-channel head coil. Imaging parameters were as follows: TR = 2000 ms, echo time (TE) = 30 ms, flip angle = 90°, field of view (FOV) = 240 × 240 mm, acquisition matrix size = 80 × 80, acquisition resolution = 3.0 × 3.0 × 4.5 mm, number of slices = 31, slice thickness = 4.5 mm with no gap between slices, number of scans = 176, SENSE factor = 2.0, and scan time = 6 minutes. In addition, sagittal structural threedimensional (3D) T1-weighted images (T1WI) were acquired with a magnetization-prepared rapid acquisition of gradient echo sequence to create templates for anatomical brain image registration. Acquisition parameters were as follows: TR = 8.1 ms, TE = 3.7 ms, flip angle = 8°, FOV = 236 × 236 mm2, and voxel size = 1 × 1 × 1 mm3. Furthermore, T2-weighted axial images and 3D FLAIR images with 1-mm isotropic voxels were acquired to screen for gross structural abnormalities in the temporal bones or brain.
Preprocessing was performed using statistical parametric mapping software (SPM8; http://www.fil.ion.ucl.ac.uk/spm/). The first 4 images of BOLD fMRI were discarded due to initial signal instability and subject adaptation. The remaining raw BOLD MRIs were realigned to the first image from each session to correct for interscan head motion. Mean individual motion was calculated for each subject as the average of the 6 realignment parameters estimated by SPM. The mean realigned fMRI of each subject was co-registered with 3D T1WI to transform the fMRI data to the normal template space. 3D T1 volumetric images were segmented into gray matter (GM), white matter (WM), cerebrospinal fluid, and non-brain tissue. The DARTEL algorithm from SPM8 was used to create a subject population template by non-linearly registering all subject-specific GM/WM templates. The transformations obtained for T1 to Montreal Neurological Institute (MNI) population template registration were concatenated to transform the fMRI images to the MNI atlas space. Finally, spatial smoothing was applied by convolving each fMRI volume with the Gaussian kernel having a full width at half maximum of 8 × 8 × 8 mm.
Using the smoothed rs-fMRI data, an independent component analysis (ICA) was performed using the group ICA from the fMRI toolbox (GIFT; http://icatb.sourceforge.net) (21). Analyses of rs-fMRI using GIFT were processed with the recommended settings of intensity normalization, ICA3 group dimension reduction and the Infomax algorithm, to estimate the number of components in each subject's dataset. Twenty IC maps were identified based on the similarity between localized spatial connectivity patterns and commonly reported resting-state networks in cortical regions (22). Default mode network (DMN) and AN were selected from 20 sets of IC maps in each group for further analysis. The z-score for every voxel was estimated by normalizing each voxel's intensity with respect to intensity of all the voxels in the independent component. We used the term "FC" to refer to the intensity (z-score) of the resting-state network signal. The regions of interest (ROIs) were defined on the average DMN and AN maps calculated using all subjects. The DMN ROIs were located at the posterior cingulate/precuneus cortices (PCC), right/left inferior parietal cortex (rIPC, lIPC), and medial prefrontal/anterior cingulate cortices (mPFC). Components of the mean DMN for all subjects were extracted from the ROI. The AN ROIs were located at the encompassed right/left superior temporal gyrus and insula (rAC, lAC). Components of the mean AN for all subjects were extracted. Mean z-scores of FC for each component of the DMN and the AN were extracted from the individual FC maps using ROIs from previous processing.
One-way analysis of variance (ANOVA) was used to assess between-group differences in clinical information, followed by post-hoc analysis of only those variables which showed significant heterogeneity across groups. The Kruskal-Wallis test was used for analysis of non-parametric data. p-values less than 0.05 were considered statistically significant.
ANOVA and the post hoc test were also used to assess between- group differences in FC of the DMN and the AN in each ROI. z-scores of rAC and lAC were compared using a paired ttest to assess the lateralization of auditory cortices within each group. p-values less than 0.05 were considered statistically significant.
The AN and DMN maps were compared between groups on a voxel-wise basis with the one-way ANCOVA in SPM8 after adjusting for subject age and sex. Statistical inferences were made with a voxel-level threshold of p < 0.01 after family-wise error correction for multiple comparisons and a 10-voxel clustering threshold.
There were no significant differences in demographics between the control group and the tinnitus groups. In addition, there were no significant differences in BDI, tinnitus pitch matching, duration of tinnitus, THI, or basic demographics among the three tinnitus groups (Table 1).
There were significant differences between groups on comparing ROI analysis results for the auditory cortices. z-scores for the rAC in the Rt-/Lt-T groups (1.97 ± 0.31, 1.72 ± 0.36, respectively) were significantly higher than those in the control and Bil-T groups (1.42 ± 0.29, 1.37 ± 0.32, respectively). Analysis of z-scores for the lAC showed a difference only between the Rt-T and control groups (1.35 ± 0.21 vs. 1.65 ± 0.23) (Table 2, Fig. 1A). Lateralization of the auditory cortex was assessed by comparing the rAC and lAC, and the Rt-/Lt-T groups had significantly higher z-scores in the rAC than in the lAC, while the control and Bil-T groups had significantly higher z-scores in the lAC than in the rAC (Table 2, Fig. 1B).
Abbreviations of brain parcellation are summarized in Table 3. The result of voxel-based analysis showed that the Rt-T group had common clusters with higher FC in the right middle temporal gyrus (MTG), MTG temporal pole, and precentral gyrus (PRECG) compared to the other three groups. In addition to these common clusters, the Rt-T group had clusters with higher FC in the right hippocampus, superior frontal gyrus (SFG), inferior frontal gyrus, orbital, and left MTG compared to the Bil-T and Lt-T groups. The Bil-T group had multiple higher clusters in the left hemisphere, including middle frontal gyrus (MFG), PRECG, supramarginal gyrus (SMG), inferior parietal gyrus (Fig. 2, Table 4), compared with the Rt-T group.
The ROI analysis showed significant differences in FC of the DMN among the four groups. The post hoc test showed that FC of the PCC in the Rt-T group was significantly lower than that in the other three groups, and FC of the mPFC in the Rt-T group was significantly higher than that in the other 3 groups. rIPC values in the three tinnitus groups were significantly higher than that in the control group. Analysis of lIPC did not show any difference between groups (Table 2, Fig. 1C).
In the voxel-based comparison of DMN maps, the Rt-T group had three regions of higher FC compared to the control group. Clusters with higher FC in the Rt-T group were located in the Rt SFG and Rt MFG, and the Rt MTGP and middle occipital gyrus. The Rt-T group also had higher FC clusters in the Rt medial superior frontal gyrus (SFGM) than the Bil-T group. The Rt-T group had lower FC in the Lt MFG than the Lt-T group (Fig. 3, Table 4).
The objective of the current study was to investigate FC differences according to laterality of tinnitus. ROI analysis of FC revealed that the two monolateral tinnitus groups had right dominance of the AN, whereas the control and Bil-T groups had left dominance. A voxel-based group comparison of tinnitus groups showed significantly different activation in the AN and the DMN between groups. These are novel findings which illustrate how the FC of resting-state in tinnitus differs according to tinnitus laterality.
Several previous studies analyzed the difference in rs-fMRI among tinnitus patients compared to normal control groups, but most of the studies did not consider lateralization. These studies reported that increased FC caused by tinnitus occurred in multiple extra-auditory regions that were not identified in the AN of the normal cohort. Kim et al. (7) observed increased FC predominantly in the left amygdala and in the mPFC of the tinnitus patients. Maudoux et al. (6) found that the AN in the tinnitus group was comprised of multiple extra-auditory regions, in addition to auditory cortices encompassing all of the previously mentioned areas such as bilateral superior temporal gyrus, SMG, and medial postcentral gyrus. These findings suggest that tinnitus is associated with modified cortical and subcortical FC (6), which is supported by a previous graphical connectivity analysis of FC (11). However, after correcting for multiple statistical comparisons in the analysis, this modification was no longer observed (8). In the voxel-wise comparison in this study, only the Rt-T group showed clusters with increased FC of the AN compared to the normal controls. In the Rt-T group, the right MTG and temporopolar area had increased FC, which is not consistent with previous reports of extra-auditory clusters. This inconsistency between studies could be due to differences in analysis methods, unrevealed variables, or heterogeneity in cohorts. Previous functional neuroimaging studies have suggested that the MTG is involved in several cognitive processes including language and semantic memory processing (2324). The temporopolar area, along with its connections to the hippocampus and amygdala, is considered a paralimbic region. Previous tinnitus studies have reported changes in the relationship between the limbic system and the auditory cortex.
Interestingly, in the present study, the FC of the AN differed significantly according to the tinnitus group. This suggests that modification of FC in the AN could differ according to the laterality of tinnitus. Thus, the laterality of tinnitus may have affected the results of previous studies, resulting in discordance between the present study and past research. Therefore, future studies should control for lateralization of tinnitus.
Although many studies have used task-based fMRI or other modalities, it is uncertain whether lateralized tinnitus is related to changes in neural activity on the ipsilateral or contralateral side. A previous study with task-based fMRI demonstrated that fMRI activation was lateralized towards the side of perceived tinnitus in the primary AC and IC in patients with Rt-T and in the medial geniculate body (MGB) in patients with Lt-T (16). PET studies showed that left-sided metabolic activation of the primary auditory cortex was observed in tinnitus patients irrespective of tinnitus laterality (1725). The present study showed over-connectivity in the right auditory and periauditory cortices in monolateral tinnitus, regardless of laterality. This finding implies that the right temporal gyrus may play a more critical role than the left temporal gyrus in creating tinnitus noise or in the perception of tinnitus. A previous PET study tested asymmetry in the auditory cortex in tinnitus patients and observed asymmetric metabolic activity in the tinnitus groups compared to the controls (20). The authors of that study observed left hyperactivity in the control group and right-dominant activity in tinnitus patients, which are similar to the findings presented here.
On comparison of the auditory cortex, the Rt-T group showed over-connectivity in the right frontal cortex (precentral, inferior orbitofrontal, SFG) compared to normal controls and other tinnitus groups. Abnormalities in the frontal cortex which could account for tinnitus have been identified in a previous neuroimaging study (26). The frontal cortex is hypothesized to play an important role in compensation, and psychological symptom in tinnitus. Different connectivities of the AN in the frontal cortex according to the location of tinnitus indicates that different localization of tinnitus perception may account for the difference in connectivity among collaborating regions of the auditory cortex in the frontal cortex.
Several recent rs-fMRI studies using the seeding method have found alterations in networks associated with emotion and attention, indicating dependence on tinnitus symptoms. Schmidt et al. (13) found that a change in FC in the limbic system in tinnitus may result in alteration of other resting FCs such as those in the DMN or the dorsal attention network. Another researcher hypothesized that tinnitus causes changes in the organization of sensory networks and interferes with the networks of attention (9). However, there are significantly fewer studies about tinnitus and non-auditory neural networks compared to studies that focused on tinnitus and AN. In the present study, only the Rt-T group had several clusters with increased FC, which were found in the right SFG and MFG; several clusters had different FCs between the Rt-T and Lt-/Bil-T groups. These results imply that chronic tinnitus may be related to aberrant functioning of the DMN, and that differences in the laterality of tinnitus may be related to non-ANs. Future research should explore why the Rt-T group primarily showed changes in the AN and the DMN.
There were some limitations to this work. First, this study was limited by the fact that rs-fMRI was compared between tinnitus patients with hearing loss and a control group without hearing loss. Further research that includes a control group with hearing loss is required to improve our understanding. Second, the imaging protocol may have also affected the results. Finally, this auditory fMRI study was limited by the presence of MR scanner noise.
This study found alterations in the auditory and default mode functional networks in tinnitus subjects at rest, and these changes appeared to be related to laterality. Therefore, laterality should be considered when analyzing rs -fMRI in tinnitus subjects. We observed significant changes in the auditory cortex according to laterality of tinnitus. This information may help clarify the neural mechanism of tinnitus and improve localized treatment. Unfortunately, not all of the results reported in this study are statistically significant. This is likely to be due to the heterogeneity in the patient group, which is the primary limitation of this study.
Figures and Tables
Fig. 1
Bar graph comparing functional connectivity between tinnitus groups.
A. Bar graph comparing connectivity of auditory cortices in the auditory network according to laterality of tinnitus. Connectivity of rAC in the monolateral tinnitus groups is significantly higher than that in the normal control and bilateral tinnitus groups. Connectivity of lAC is higher in the normal control and Bil-T groups than in the monolateral tinnitus groups.
B. Bar graph showing asymmetric dominance of AN in each group. The two monolateral tinnitus groups show right dominance in FC of the AN, while the bilateral tinnitus and control groups show left dominance.
C. Bar graph comparing the connectivity of major clusters in the default mode network according to laterality of tinnitus. The Rt-T group shows significantly different FCs in the PCC and mPFC compared with the other three groups. All three tinnitus groups have higher connectivity in rIPC than the normal controls.
AN = auditory network, Bil-T = bilateral tinnitus, CN_R_L_B = control, right tinnitus, left tinnitus, bilateral tinnitus, FC = functional connectivity, lAC = left superior temporal gyrus and insula, lIPC = left inferior parietal cortex, Lt-T = left-sided tinnitus, mPFC = medial prefrontal/anterior cingulate cortices, PCC = posterior cingulate/precuneus cortices, rAC = right superior temporal gyrus and insula, rIPC = right inferior parietal cortex, Rt-T = right-sided tinnitus
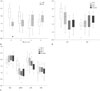
Fig. 2
Between-group functional connectivity comparison in the AN.
A. Comparison of the Rt-T group vs. control group. Clusters in red signify higher FC in the Rt-T group than in the control group.
B. Comparison of the Rt-T group vs. the Lt-T group. Clusters in red signify higher FC in the Rt-T group than in the Lt-T group.
C. Comparison of the Rt-T group vs. the Bil-T group. Clusters in red signify higher FC in the Rt-T group than in the Bil-T group, and clusters in blue signify higher FC in the Bil-T group than in the Rt-T group.
AN = auditory network, Bil-T = bilateral tinnitus, FC = functional connectivity, Lt-T = left-sided tinnitus, Rt-T = right-sided tinnitus
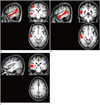
Fig. 3
Between-group functional connectivity comparison in the DMN.
A. Comparison of the Rt-T group vs. control group. Clusters in red signify higher FC in the Rt-T group than in the control group.
B. Comparison of the Rt-T group vs. Lt-T group. Clusters in blue signify higher FC in the Lt-T group than in the Rt-T group.
C. Comparison of the Rt-T group vs. the Bil-T group. Clusters in red signify higher FC in the Rt-T group than in the Bil-T group.
Bil-T = bilateral tinnitus, DMN = default mode network, FC = functional connectivity, Lt-T = left-sided tinnitus, Rt-T = right-sided tinnitus
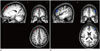
Table 1
Information of Dermographics and Otologic Examination
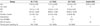
Table 2
ROI Measurement of Z-Score from Common Clusters in AN and DMN
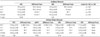
AN = auditory network, Bil-T = bilateral tinnitus, DMN = default mode network, lAC = left superior temporal gyrus and insula, lIPC = left inferior parietal cortex, Lt-T = left-sided tinnitus, mPFC = medial prefrontal/anterior cingulate cortices, PCC = posterior cingulate/precuneus cortices, rAC = right superior temporal gyrus and insula, rIPC = right inferior parietal cortex, ROI = region of interest, Rt-T = right-sided tinnitus
Table 3
Abbreviations of Cortical Parcellation
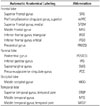
Table 4
Voxel-Wised Analysis Results
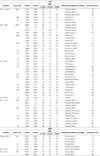
Acknowledgments
This study was supported by a Bayer-Schering Radiological Research Fund of the Korean Society of Radiology for 2011.
References
1. Eggermont JJ, Roberts LE. The neuroscience of tinnitus. Trends Neurosci. 2004; 27:676–682.
2. Han BI, Lee HW, Kim TY, Lim JS, Shin KS. Tinnitus: characteristics, causes, mechanisms, and treatments. J Clin Neurol. 2009; 5:11–19.
3. Adjamian P, Sereda M, Hall DA. The mechanisms of tinnitus: perspectives from human functional neuroimaging. Hear Res. 2009; 253:15–31.
4. Schlee W, Mueller N, Hartmann T, Keil J, Lorenz I, Weisz N. Mapping cortical hubs in tinnitus. BMC Biol. 2009; 7:80.
5. Schlee W, Weisz N, Bertrand O, Hartmann T, Elbert T. Using auditory steady state responses to outline the functional connectivity in the tinnitus brain. PLoS One. 2008; 3:e3720.
6. Maudoux A, Lefebvre P, Cabay JE, Demertzi A, Vanhaudenhuyse A, Laureys S, et al. Auditory resting-state network connectivity in tinnitus: a functional MRI study. PLoS One. 2012; 7:e36222.
7. Kim JY, Kim YH, Lee S, Seo JH, Song HJ, Cho JH, et al. Alteration of functional connectivity in tinnitus brain revealed by resting-state fMRI? A pilot study. Int J Audiol. 2012; 51:413–441.
8. Davies J, Gander PE, Andrews M, Hall DA. Auditory network connectivity in tinnitus patients: a resting-state fMRI study. Int J Audiol. 2014; 53:192–198.
9. Burton H, Wineland A, Bhattacharya M, Nicklaus J, Garcia KS, Piccirillo JF. Altered networks in bothersome tinnitus: a functional connectivity study. BMC Neurosci. 2012; 13:3.
10. Ueyama T, Donishi T, Ukai S, Ikeda Y, Hotomi M, Yamanaka N, et al. Brain regions responsible for tinnitus distress and loudness: a resting-state FMRI study. PLoS One. 2013; 8:e67778.
11. Maudoux A, Lefebvre P, Cabay JE, Demertzi A, Vanhaudenhuyse A, Laureys S, et al. Connectivity graph analysis of the auditory resting state network in tinnitus. Brain Res. 2012; 1485:10–21.
12. Wineland AM, Burton H, Piccirillo J. Functional connectivity networks in nonbothersome tinnitus. Otolaryngol Head Neck Surg. 2012; 147:900–906.
13. Schmidt SA, Akrofi K, Carpenter-Thompson JR, Husain FT. Default mode, dorsal attention and auditory resting state networks exhibit differential functional connectivity in tinnitus and hearing loss. PLoS One. 2013; 8:e76488.
14. Lanting CP, de Kleine E, Langers DR, van Dijk P. Unilateral tinnitus: changes in connectivity and response lateralization measured with FMRI. PLoS One. 2014; 9:e110704.
15. Boyen K, de Kleine E, van Dijk P, Langers DR. Tinnitus-related dissociation between cortical and subcortical neural activity in humans with mild to moderate sensorineural hearing loss. Hear Res. 2014; 312:48–59.
16. Smits M, Kovacs S, de Ridder D, Peeters RR, van Hecke P, Sunaert S. Lateralization of functional magnetic resonance imaging (fMRI) activation in the auditory pathway of patients with lateralized tinnitus. Neuroradiology. 2007; 49:669–679.
17. Arnold W, Bartenstein P, Oestreicher E, Römer W, Schwaiger M. Focal metabolic activation in the predominant left auditory cortex in patients suffering from tinnitus: a PET study with [18F]deoxyglucose. ORL J Otorhinolaryngol Relat Spec. 1996; 58:195–199.
18. De Ridder D, De Mulder G, Verstraeten E, Van der Kelen K, Sunaert S, Smits M, et al. Primary and secondary auditory cortex stimulation for intractable tinnitus. ORL J Otorhinolaryngol Relat Spec. 2006; 68:48–54. discussion 54-55.
19. van der Loo E, Congedo M, Vanneste S, Van De Heyning P, De Ridder D. Insular lateralization in tinnitus distress. Auton Neurosci. 2011; 165:191–194.
20. Geven LI, de Kleine E, Willemsen AT, van Dijk P. Asymmetry in primary auditory cortex activity in tinnitus patients and controls. Neuroscience. 2014; 256:117–125.
21. Calhoun VD, Adali T, Pearlson GD, Pekar JJ. A method for making group inferences from functional MRI data using independent component analysis. Hum Brain Mapp. 2001; 14:140–151.
22. Damoiseaux JS, Rombouts SA, Barkhof F, Scheltens P, Stam CJ, Smith SM, et al. Consistent resting-state networks across healthy subjects. Proc Natl Acad Sci U S A. 2006; 103:13848–13853.
23. Chao LL, Haxby JV, Martin A. Attribute-based neural substrates in temporal cortex for perceiving and knowing about objects. Nat Neurosci. 1999; 2:913–919.
24. Tranel D, Damasio H, Damasio AR. A neural basis for the retrieval of conceptual knowledge. Neuropsychologia. 1997; 35:1319–1327.
25. Langguth B, Eichhammer P, Kreutzer A, Maenner P, Marienhagen J, Kleinjung T, et al. The impact of auditory cortex activity on characterizing and treating patients with chronic tinnitus--first results from a PET study. Acta Otolaryngol Suppl. 2006; (556):84–88.
26. Schecklmann M, Landgrebe M, Poeppl TB, Kreuzer P, Männer P, Marienhagen J, et al. Neural correlates of tinnitus duration and distress: a positron emission tomography study. Hum Brain Mapp. 2013; 34:233–240.