Abstract
The incidence of thyroid cancer is growing the fastest among all cancers in the United States, especially in women. The number of patients with thyroid neoplasm is part of an even larger number of patients who often need to undergo an operation to exclude a cancer diagnosis. While differentiated thyroid cancer (papillary thyroid cancer and follicular thyroid cancer) accounts for most cases of thyroid cancer and has a relatively good prognosis, effective treatments for patients with de-differentiated and anaplastic thyroid cancer are still gravely needed. Despite progress in the identification of genetic changes in thyroid cancer, the impact of aberrant epigenetic alterations on thyroid cancer remains to be fully elucidated. Understanding of the roles of epigenetic changes in thyroid cancer could open new opportunities for the identification of innovative molecular targets for novel treatment modalities, especially for anaplastic thyroid cancer for which treatment is very limited. This article briefly reviews the studies that exemplify the potential for and promise of using epigenetic regulators in the treatment of thyroid cancer.
Cancer is a malignancy with many genetic and epigenetic alterations. Genetic changes involve the heritable alterations of DNA sequences, while epigenetic alterations are the heritable changes to gene expression that do not involve changes of nucleotide sequences. Epigenetic modifications can change the transcription and translation of target genes to increase or decrease their functional levels. A well-known example is the DNA methylation by the addition of methyl groups to CpG dinucleotides in DNA, frequently resulting in gene silencing [12]. Other well-studied epigenetic modifications that affect gene expression include the post-translational modification of histone proteins by acetylation, methylation, phosphorylation, ubiquitylation, sumoylation, proline isomerization, and adenosine diphosphate-ribosylation [3]. These epigenetic changes play critical roles in responding to dynamic transcriptional changes in cells during normal development and differentiation. However, recent studies have shown that in addition to genetic aberrations, aberrant epigenetic alterations contribute to cancer development and progression.
Thyroid cancers are the most common malignancies of the endocrine system. Based on differentiation status, thyroid cancer derived from follicular epithelial cells is classified into three major categories: well-differentiated thyroid carcinoma, including follicular and papillary thyroid carcinoma; poorly differentiated thyroid carcinoma; and anaplastic thyroid carcinoma (ATC). While the overall 5-year survival of well-differentiated thyroid carcinoma is approximately 85% to 90%, ATC represents one of the most lethal cancers in humans. Effective treatment for ATC is very limited, and patients with ATC rarely survive beyond 6 months. Although the genetic aberrations in differentiated thyroid cancer (DTC) and ATC have been intensively studied, the epigenetic profiling of differentiated and undifferentiated thyroid cancer (UDTC) has just begun to be unraveled. Gene expression profiling of DTC has indicated that alterations in DNA methylation, and histone modifications, long non-coding RNAs and microRNAs could contribute to tumor development and progression [4]. Genomic and transcriptomic features of ATC have recently been identified [5]. In addition to the previously known genetic aberrations (e.g., TP53, the TERT promoter, and BRAF), mutations in genes involved in chromatin remodeling, such as SWI/SNF (switch/sucrose non-fermentable) subunits and histone methyltransferases, were also detected. Mutations in genes important for chromatin functions would lead to aberrant epigenetic alterations, impacting thyroid carcinogenesis. These findings underscore the critical role of epigenetic regulation in thyroid tumor biology and in clinical relevance. This review will focus on the recent advances in assessing epigenetic modifications as a potential therapeutic approach in DTC and ATC.
Gene transcription is regulated by chromatin structure. The open conformation of chromatin facilitates gene transcription, whereas tightly packed chromatin conformation deters transcription. One mechanism that affects the chromatin conformation is DNA methylation, which occurs mainly in CpG islands. That aberrant DNA methylation of tumor-suppressor genes occurs during thyroid carcinogenesis is exemplified by the studies of several tumor suppressors using the gene-by-gene approach. Studies have shown reduced or lost expression of the thyroid hormone receptor β gene (THRB) in many cancers. Analysis of the expression of the THRB gene found it to be 90% lower in DTC samples than in the normal controls. Further, the extent of decreased THRB gene expression is inversely correlated with the severity in the progression of DTC [6]. Consistent with the mRNA expression patterns, TRβ protein levels are lower in DTC samples than in the normal controls. Analysis by methylation-specific polymerase chain reaction (PCR) showed that the extent of the THRB gene promoter methylation is greater in thyroid cancer tissues than in normal controls [6]. These findings suggest that TRβ acts as a tumor suppressor in DTC and that aberrant DNA methylation of the THRB gene contributes to thyroid cancer progression. These results prompted the use of demethylation agents to re-express the THRB gene to revert the thyroid cancer phenotypes. Human FTC-236 (follicular thyroid cancer) cells were treated with demethylating agents 5-aza-CdR and zebularine to induce the expression of the THRB gene. Remarkably, treatment with either aza-CdR or zebularine led to re-expression of the THRB gene and the reduction of tumor cell proliferation and migration. In addition, FTC-236 cells stably expressing TRβ exhibit lower cell proliferation and migration through inhibition of β-catenin signaling pathways than do FTC-236 cells without TRβ. In mouse xenograft models, demethylating agent 5-aza-CdR was shown to inhibit growth of tumors induced by FTC-236 cancer cells [6]. These findings indicate that TRβ is a tumor suppressor and that epigenetic reactivation of its expression could be tested as a potential therapeutic target in DTC.
Another example of epigenetic modification by de-methylation to re-express a tumor suppressor in DTC was reported by Moraes et al. [7]. ABL-interactor member 3 (ABI3) was shown to be a tumor suppressor that plays important roles in the malignant transformation of thyroid tumors [8]. The expression of ABI3 is frequently reduced or lost in follicular thyroid carcinomas. Ectopic expression of ABI3 inhibited cell proliferation, invasion, migration, and delayed cell cycle progression in thyroid cancer cells [8]. The transcription silencing of ABI3 in thyroid cancer occurs via methylation of specific CpG sites located within the ABI3 promoter [7]. Treatment of four follicular thyroid carcinoma cell lines with 5-aza-dC induced demethylation of a specific region of the ABI3 promoter and restored ABI3 expression. In contrast, 5-aza-dC treatment did not restore ABI3 expression in a non-thyroid cell line, suggesting a tissue-specific regulation. In most thyroid carcinoma samples, eight CpG sites were found to be located within the ABI3 promoter, and the degree of methylation correlated with the extent of expression [7]. These findings suggest that epigenetic de-methylation to re-express the ABI3 gene could be potentially beneficial for thyroid cancer.
Post-translational modification of histones is also known to play critical roles in tumorigenesis [9]. The effect of histone acetylation and de-acetylation on gene transcription has been well studied. The two enzymes, histone acetyltransferase (HAT) and histone deacetylase (HDAC), responsible for the reversible acetylation-deacetylation changes could be dis-regulated during carcinogenesis. Thus, HAT and HDAC have been evaluated as targets for novel anti-cancer drugs [10]. Indeed, HDAC inhibitors have been evaluated in the clinical trials for the treatment of radioiodine-refractory metastatic non-medullary thyroid carcinomas. While the trials on the efficacy of other HDAC inhibitors are still ongoing, the phase II trials of Vorinostat, also known as suberanilhydroxamic acid (SAHA) [11], and romidepsin [12] have been completed. For the Vorinostat trial, no patients achieved a partial or complete response. For the romidepsin trial, except for the preliminary signs of in vivo reversal of radioactive iodine-refractory resistance in two patients, no major responses were detected. In both trials, clinical grade 3 to 5 adverse events were observed. While the phase II trial for both inhibitors suggested that single-agent treatment of these two inhibitors with other agents could be effective, this possibility needs to be tested in future studies.
The fact that these two HDAC inhibitors were not effective as a single agent in the trial studies raised a question as to whether the patients were resistant to SAHA treatment. The molecular basis of SAHA resistance has been probed in two mouse models of metastatic thyroid cancer: THRBPV/PV and THRBPV/PVPten+/− mice [13]. In both mouse models, thyroid cancer is driven by overactivation of phosphoinositide 3-kinase (PI3K)-AKT signaling. The THRBPV/PVPten+/− mice, however, exhibit more aggressive cancer progression owing to haplodeficiency of the Pten tumor suppressor gene. SAHA treatment had no apparent effects on thyroid cancer progression in THRBPV/PV mice. Unexpectedly, however, thyroid cancer progressed more aggressively in THRBPV/PVPten+/− mice treated with SAHA than in those without SAHA treatment. Molecular analysis showed SAHA treatment further activates the PI3K-AKT signaling to increase proliferation and invasion of tumor cells. Digital PCR analysis of genomic DNAs indicated that the wild-type allele of the Pten gene was progressively lost during thyroid carcinogenesis of SAHA-treated THRBPV/PV Pten+/− mice. This SAHA-induced loss of the Pten gene results in promoting further aggressive thyroid carcinogenesis. The paradoxical actions of SAHA are reminiscent of the outcome of the clinical trial of 19 patients with advanced thyroid cancer [11] in that no patient achieved a partial or complete response. Trials for some patients were terminated owing to clinical progression of the disease, suggesting the development of resistance to SAHA treatment in patients. Resistance to HDAC inhibitors has been suggested to involve aberrant expression and modifications of signaling molecules, such as high-level expression of thioredoxin, overactivation of prosurvival BCL-2 (B-cell lymphoma 2), or constitutive activation of STAT (signal transducer and activator of transcription) proteins [14151617]. In the clinical trial of SAHA in 19 patients [11], it was not clear what regulators were aberrantly induced and/or which signaling molecules were aberrantly activated by SAHA treatment; thus, leading to resistance to SAHA and/or progression of advanced thyroid cancer. However, in a mouse model of thyroid cancer (THRBPV/PV Pten+/− mice) in which the cancer progression is driven by the activation of PI3K-AKT signaling, SAHA treatment induces the loss of the Pten gene expression. These findings raise the possibility that SAHA could change the copy number of tumor suppressors, depending on the cellular context. HDAC inhibitors have been shown to be beneficial in clinical trials for other cancer types, especially in combination with other therapeutics [18]. Still, studies of the efficacy of HDAC inhibitors in thyroid cancer are limited. In view of the findings that SAHA treatment could induce loss of a tumor suppressor (the Pten gene) in a mouse model of aggressive follicular thyroid cancer, caution is warranted if SAHA or its related HDAC inhibitors are under consideration for therapeutics in thyroid cancer in which cancer progression is driven by aberrant activation of PI3K-AKT signaling.
ATC is a very aggressive thyroid cancer for which the treatment modalities are very limited. In searching for innovative therapeutic targets, a novel class of inhibitors for the bromodomain and extra-terminal (BET) proteins has been tested in ATC. The BET family of bromodomain proteins (BRDs) is composed of four members in humans (BRD2, BRD3, BRD4, and BRDT). BET proteins act as epigenetic readers and bind to acetylated lysine residues on the tails of histones H3 and H4, regulating gene transcription. Over the past several years, the BET epigenetic readers have become the main targets for drug therapy. Selective small-molecule compounds capable of inhibiting BET proteins have been explored for the treatment of human disorders such as cancer, atherosclerosis, cardiovascular, and inflammatory diseases [19].
A BET inhibitor, JQ1, was shown to be effective in arresting growth in hematological malignancies and solid tumors such as glioblastoma, NUT (nuclear protein of the testis) midline carcinoma, lung, and prostate cancers [2021222324]. BRD4, a target of JQ1, is generally involved in the transcription of active genes and is associated with most active enhancers and promoters (termed super-enhancers) in tumor cells [25]. JQ1 causes a selective loss of BRD4 at super-enhancers, resulting in preferential loss of transcription at super-enhancer-associated genes such as the MYC oncogene [25]. Aberrantly elevated MYC was found in human ATC [26]. In the tumors of a mouse model of ATC (THRBPV/PVKras_G12D mice [27]), overexpression of MYC was found to propel thyroid tumor growth [27]. This THRBPV/PV KrasG12D mouse co-expresses germline mutations of a thyroid hormone receptor β (TRβPV) and KRASG12D in the thyroid. THRBPV/PVKrasG12D mice exhibited capsular invasion, vascular invasion, metastasis, and high frequency of anaplastic foci with complete loss of normal thyroid follicular morphology [27]. The efficacy of JQ1 in suppressing ATC was assessed in THRBPV/PV KrasG12D mice [28]. JQ1 markedly inhibited thyroid tumor growth and prolonged survival of these mice. During 10-week treatment, none of JQ1-treated mice died while more than half of vehicle-treated mice died (Fig. 1A). JQ1 inhibited thyroid tumor growth of these mice (Fig. 1B). Fig. 1C shows a representative example of reduced tumor size in mice treated with JQ1 as compared with the larger tumor size from mice treated with vehicle (Fig. 1C, thyroid tumors indicated by arrows).
JQ1 suppressed the MYC transcription program by inhibiting mRNA expression of Myc, Ccnd1, and other MYC downstream target genes. JQ1-suppressed Myc expression was accompanied by chromatin remodeling as evidenced by increased expression of histones and hexamethylene bisacetamide inducible 1, a suppressor of RNA polymerase II transcription elongation. JQ1 decreased MYC protein abundance in thyroid tumors and attenuated the cyclin D1-CDK4-Rb-E2F3 signaling to decrease tumor growth. JQ1 inhibited the recruitment of BDR4 (bromodomain-containing protein 4) to the promoter complex of the Myc and Ccnd1 genes in rat thyroid follicular PCCL3 cells, resulting in decreased MYC expression at the mRNA and protein levels to inhibit tumor cell proliferation. These preclinical findings suggest that BET inhibitors could serve as novel therapeutics to reduce ATC tumor growth.
That BET inhibitors could be a promising therapeutic strategy for ATC was further supported by the studies of efficacy of JQ1 in four human ATC cells [26]. The four ATC human cell lines, designated THJ-11T, THJ-16T, THJ-21T, and THJ-29T, were established from human primary ATC tumors [29]. They were shown to harbor complex genetic alternations. In addition to copy number gains and losses in various genes, THJ-11T cells express KRASG12V mutation; THJ-16T cells express PI3KE454K, TP53, and Rb mutations; THJ-21T cells express BRAFV600E, TP53, and Rb mutations; and THJ-29T cells express Rb mutations [29]. These authenticated cell lines have been used by investigators as model cell lines to interrogate the functional consequences of these mutations and to identify potential molecular targets for treatment [3031]. Consistent with the findings from THRBPV/PVKrasG12D mice, JQ1 markedly inhibited proliferation of four ATC cell lines by suppression of MYC and elevation of p21 and p27 to decrease phosphorylated Rb to delay cell cycle progression from the G0/G1 phase to the S phase. JQ1 blocked cell invasion by attenuating epithelial mesenchymal transition signals with decreased TWIST1, snail, and slug. These cell-based studies were further confirmed in xenograft studies in that the size and rate of tumor growth was inhibited by JQ1 via inhibition of p21-Cyclin/CDK-Rb-E2F signaling [26]. The findings from human ATC cell lines and the preclinical mouse model of ATC indicate that BET inhibitors could be a potential novel treatment modality for human ATC. With additional second generations of BET inhibitors being developed, this novel class of BET inhibitors hold great promise for treating ATC.
Recent advances in the understanding of epigenetic alterations on cancer biology have opened exciting possibilities in identifying chromatin modulators as new therapeutics for a variety of cancers. Studies in mouse models and cell lines from human anaplastic thyroid tumors indicate that targeting epigenetic modifications may present a novel effective approach to treat ATC. Whether the suppression effects of BET inhibitors (e.g., JQ1) on the growth of ATC could be long-lasting without development of resistance is currently unknown. With the newer and more effective BET inhibitors being developed and tested, resistance could be minimized. In addition, targeting epigenetic regulators in a combination with inhibitors in other pathways (e.g., kinase inhibitors) could lead to better or even curative treatment. The chromatin landscape is dynamically shaped by versatile epigenetic modulators. Targeting chromatin epigenetic modifiers for therapies of ATC holds great promise.
Figures and Tables
Fig. 1
JQ1 treatment is effective in prolonging survival (A), reducing tumor weight (B) and tumor size (C) of THRBPV/PVKrasG12D mice. (A) JQ1 treatment prolongs survival of THRBPV/PVKrasG12D mice. The Kaplan-Meier survival curves for THRBPV/PVKrasG12D mice treated with vehicle (n=10) or JQ1 (n=10) for 10 weeks. (B) JQ1 treatment reduces thyroid tumor weight of THRBPV/PVKrasG12D mice. Thyroid weight was reduced by 57.7% in the mice treated with JQ1. (C) JQ1 treatment reduces thyroid tumor size of THRBPV/PVKrasG12D mice. Representative thyroid tumors from vehicle-treated mice indicated by the arrow (panel a) or from JQ1-treated mice indicated by the arrow (panel b) are shown.
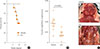
ACKNOWLEDGMENTS
We regret any reference omissions due to length limitation. We wish to thank all colleagues and collaborators who have contributed to the work described in this review. The research described in this review by the authors and their colleagues at the National Cancer Institute was supported by the Intramural Research Program of the Center for Cancer Research, National Cancer Institute, National Institutes of Health.
References
1. Deaton AM, Bird A. CpG islands and the regulation of transcription. Genes Dev. 2011; 25:1010–1022.
2. Jones PA, Baylin SB. The fundamental role of epigenetic events in cancer. Nat Rev Genet. 2002; 3:415–428.
3. Peterson CL, Laniel MA. Histones and histone modifications. Curr Biol. 2004; 14:R546–R551.
4. Asa SL, Ezzat S. The epigenetic landscape of differentiated thyroid cancer. Mol Cell Endocrinol. 2017; 07. 12. [Epub]. DOI: 10.1016/j.mce.2017.07.012.
5. Landa I, Ibrahimpasic T, Boucai L, Sinha R, Knauf JA, Shah RH, et al. Genomic and transcriptomic hallmarks of poorly differentiated and anaplastic thyroid cancers. J Clin Invest. 2016; 126:1052–1066.
6. Kim WG, Zhu X, Kim DW, Zhang L, Kebebew E, Cheng SY. Reactivation of the silenced thyroid hormone receptor β gene expression delays thyroid tumor progression. Endocrinology. 2013; 154:25–35.
7. Moraes L, Galrao AL, Rubio I, Cerutti JM. Transcriptional regulation of the potential tumor suppressor ABI3 gene in thyroid carcinomas: interplay between methylation and NKX2-1 availability. Oncotarget. 2016; 7:25960–25970.
8. Latini FR, Hemerly JP, Freitas BC, Oler G, Riggins GJ, Cerutti JM. ABI3 ectopic expression reduces in vitro and in vivo cell growth properties while inducing senescence. BMC Cancer. 2011; 11:11.
9. Chi P, Allis CD, Wang GG. Covalent histone modifications: miswritten, misinterpreted and mis-erased in human cancers. Nat Rev Cancer. 2010; 10:457–469.
10. Noureen N, Rashid H, Kalsoom S. Identification of type-specific anticancer histone deacetylase inhibitors: road to success. Cancer Chemother Pharmacol. 2010; 66:625–633.
11. Woyach JA, Kloos RT, Ringel MD, Arbogast D, Collamore M, Zwiebel JA, et al. Lack of therapeutic effect of the histone deacetylase inhibitor vorinostat in patients with metastatic radioiodine-refractory thyroid carcinoma. J Clin Endocrinol Metab. 2009; 94:164–170.
12. Sherman EJ, Su YB, Lyall A, Schoder H, Fury MG, Ghossein RA, et al. Evaluation of romidepsin for clinical activity and radioactive iodine reuptake in radioactive iodine-refractory thyroid carcinoma. Thyroid. 2013; 23:593–599.
13. Zhu X, Kim DW, Zhao L, Willingham MC, Cheng SY. SAHA-induced loss of tumor suppressor Pten gene promotes thyroid carcinogenesis in a mouse model. Endocr Relat Cancer. 2016; 23:521–533.
14. Fedier A, Dedes KJ, Imesch P, Von Bueren AO, Fink D. The histone deacetylase inhibitors suberoylanilide hydroxamic (Vorinostat) and valproic acid induce irreversible and MDR1-independent resistance in human colon cancer cells. Int J Oncol. 2007; 31:633–641.
15. Fantin VR, Loboda A, Paweletz CP, Hendrickson RC, Pierce JW, Roth JA, et al. Constitutive activation of signal transducers and activators of transcription predicts vorinostat resistance in cutaneous T-cell lymphoma. Cancer Res. 2008; 68:3785–3794.
16. Shao W, Growney JD, Feng Y, O'Connor G, Pu M, Zhu W, et al. Activity of deacetylase inhibitor panobinostat (LBH589) in cutaneous T-cell lymphoma models: defining molecular mechanisms of resistance. Int J Cancer. 2010; 127:2199–2208.
17. Lee JH, Choy ML, Marks PA. Mechanisms of resistance to histone deacetylase inhibitors. Adv Cancer Res. 2012; 116:39–86.
18. Eckschlager T, Plch J, Stiborova M, Hrabeta J. Histone deacetylase inhibitors as anticancer drugs. Int J Mol Sci. 2017; 18:E1414.
19. Radwan M, Serya R. Fragment-based drug discovery in the bromodomain and extra-terminal domain family. Arch Pharm (Weinheim). 2017; 350:e1700147.
20. Asangani IA, Dommeti VL, Wang X, Malik R, Cieslik M, Yang R, et al. Therapeutic targeting of BET bromodomain proteins in castration-resistant prostate cancer. Nature. 2014; 510:278–282.
21. Cheng Z, Gong Y, Ma Y, Lu K, Lu X, Pierce LA, et al. Inhibition of BET bromodomain targets genetically diverse glioblastoma. Clin Cancer Res. 2013; 19:1748–1759.
22. Lockwood WW, Zejnullahu K, Bradner JE, Varmus H. Sensitivity of human lung adenocarcinoma cell lines to targeted inhibition of BET epigenetic signaling proteins. Proc Natl Acad Sci U S A. 2012; 109:19408–19413.
23. Shimamura T, Chen Z, Soucheray M, Carretero J, Kikuchi E, Tchaicha JH, et al. Efficacy of BET bromodomain inhibition in Kras-mutant non-small cell lung cancer. Clin Cancer Res. 2013; 19:6183–6192.
24. Filippakopoulos P, Qi J, Picaud S, Shen Y, Smith WB, Fedorov O, et al. Selective inhibition of BET bromodomains. Nature. 2010; 468:1067–1073.
25. Loven J, Hoke HA, Lin CY, Lau A, Orlando DA, Vakoc CR, et al. Selective inhibition of tumor oncogenes by disruption of super-enhancers. Cell. 2013; 153:320–334.
26. Enomoto K, Zhu X, Park S, Zhao L, Zhu YJ, Willingham MC, et al. Targeting MYC as a therapeutic intervention for anaplastic thyroid cancer. J Clin Endocrinol Metab. 2017; 102:2268–2280.
27. Zhu X, Zhao L, Park JW, Willingham MC, Cheng SY. Synergistic signaling of KRAS and thyroid hormone receptor β mutants promotes undifferentiated thyroid cancer through MYC up-regulation. Neoplasia. 2014; 16:757–769.
28. Zhu X, Enomoto K, Zhao L, Zhu YJ, Willingham MC, Meltzer P, et al. Bromodomain and extraterminal protein inhibitor JQ1 suppresses thyroid tumor growth in a mouse model. Clin Cancer Res. 2017; 23:430–440.
29. Marlow LA, D'Innocenzi J, Zhang Y, Rohl SD, Cooper SJ, Sebo T, et al. Detailed molecular fingerprinting of four new anaplastic thyroid carcinoma cell lines and their use for verification of RhoB as a molecular therapeutic target. J Clin Endocrinol Metab. 2010; 95:5338–5347.
30. Reeb AN, Li W, Lin RY. Bioluminescent human thyrospheres allow noninvasive detection of anaplastic thyroid cancer growth and metastases in vivo. Thyroid. 2014; 24:1134–1138.
31. Marlow LA, Bok I, Smallridge RC, Copland JA. RhoB upregulation leads to either apoptosis or cytostasis through differential target selection. Endocr Relat Cancer. 2015; 22:777–792.