Abstract
Skeletal muscle possesses plasticity and adaptability to external and internal physiological changes. Due to these characteristics, skeletal muscle shows dramatic changes depending on its response to stimuli such as physical activity, nutritional changes, disease status, and environmental changes. Modulation of the rate of protein synthesis/degradation plays an important role in atrophic responses. The purpose of this review is to describe different features of skeletal muscle adaptation with various models of deceased use. In this review, four models were addressed: immobilization, spinal cord transection, hindlimb unloading, and aging. Immobilization is a form of decreased use in which skeletal muscle shows electrical activity, tension development, and motion. These results differ by muscle group. Spinal cord transection was selected to simulate spinal cord injury. Similar to the immobilization model, dramatic atrophy occurs in addition to fiber type conversion in this model. Despite the fact that electromyography shows unremarkable changes in muscle after hindlimb unloading, decreased muscle mass and contractile force are observed. Lastly, aging significantly decreases the numbers of muscle fibers and motor units. Skeletal muscle responses to decreased use include decreased strength, decreased fiber numbers, and fiber type transformation. These four models demonstrated different changes in the skeletal muscle. This review elucidates the different skeletal muscle adaptations in these four decreased use animal models and encourages further studies.
Skeletal muscle is a functional unit composed of protein structures. Through contraction and relaxation, it helps to support the body structure and creates movement. Due to skeletal muscle's innate characteristics of plasticity and adaptability, it can change its size to adapt to external and internal physiological stimuli. During growth, skeletal muscle increases in size until the organism reaches the peak of its growth period and then starts to decline. The mass and function of skeletal muscle vary depending on the organism's activity or lack thereof. For example, physical activity such as exercise influences the internal conditions of skeletal muscle and results in its increased size. In contrast, decreased use of skeletal muscle is considered to be one of the main reasons for atrophy. Environmental changes such as space flight can impact an individual's physiological conditioning and accelerate the degradation of skeletal muscle [1]. Furthermore, aging also inevitably results in decreases in skeletal muscle mass [2].
Protein turnover is an important mechanism for maintaining the balance of protein structures in the body. Modulation of the synthesis/degradation rate can result in hypertrophy or atrophy [3]. During periods of prolonged bed rest or immobilization, there is a disturbance in protein turnover, which leads to atrophic responses [3]. In addition to alterations in protein balance due to decreased use, other characteristics of skeletal muscle are changed, including specific tension and fiber type, number, and size [45678].
The purpose of this review is to describe the different features of skeletal muscle adaptation using various models of decreased use. In this review, four models that simulate conditions of decreased use condition were assessed: (1) immobilization, (2) spinal cord transection, (3) hindlimb unloading, and (4) aging.
During traumatic injury such as fracture, the injured area should be immobilized to prevent secondary damage caused by movement of that area. This model is used to mimic situations necessitating casts and elucidates the changes that occur in skeletal muscle during prolonged immobilization. To prepare this animal model, the animal's leg of interest is wrapped with a plaster bandage in the preferred joint position. One recent study tried to reproduce prior results using a surgical skin stapler [9]. Different methods acting on different immobilized joint positions can directly affect the degree of skeletal muscle atrophy [10]. During immobilization, the length of the muscle at specific fixed positions is very important. Depending on the angle of joint fixation, the agonist and antagonist muscles can be stretched or contracted [10]. Contracted muscle shows greater atrophy than stretched muscle, while skeletal muscle in the stretched position is less likely to be affected by immobilization [11]. In ankle-joint immobilization, the loss of skeletal muscle was shown to be generally greater in the extensor muscles than in the flexor muscles [71213].
The vulnerability of skeletal muscle to atrophy in a fixed position of immobilization seems to depend on fiber type [14]. Different fibers in the same skeletal muscle group show differences in size reduction between type I and type II fibers after atrophy [815]. Booth and Kelso [15] studied male rats that underwent hindlimb immobilization for a 4-week period and showed a greater reduction of type I fibers than type II fibers in the soleus muscle. In contrast to type I fibers, fast type IIx and IIb fibers were less affected by ankle-joint immobilization [78].
The effects of joint immobilization depend on how the joint and skeletal muscle are restricted with regard to maximization or minimization of the length of the fiber [16]. Two general principles of muscle activation are that atrophy in slow-twitch muscle fibers occurs before that in fast-twitch muscle fibers, and that skeletal muscles in shortened positions have greater reductions in function than lengthened position [161718].
The spinal cord transection model simulates spinal cord injury. It is easier to determine the effectiveness of muscle regeneration and functional recovery in models with complete resection.
Six months after spinal cord transection, significant reduction in the cross-sectional area (CSA) of fast- and slow-twitch fiber sizes is evident, with some atrophy in both fiber types [4]. Similar to in immobilization, conversion of fiber type from type I to type II was generally observed during spinal transection [41920]. During transection, dark-staining ATPase fiber composition increased from <1% to 45% in the soleus of a cat transection model, suggesting an increased proportion of type II fibers in the muscle [4]. Furthermore, most of the fibers in the soleus muscle reacted with fast myosin heavy chain antibody [4]. Fiber transformation has been suggested to be complete 4 months after injury [41221].
The soleus muscle in spinal cord transection models has shown dramatic increases in fusion frequency and decreases in time to peak tension [22]. This represents a slow to fast transformation, suggesting that its contractile speed and specific tension are increased after transection.
The hindlimb unloading rodent model has been widely used to simulate microgravity conditions. Since the National Aeronautics and Space Administration Ames research center adopted this model to investigate changes to skeletal muscle during space flight, it has been accepted by many researchers as a method to examine the adaptation of skeletal muscle to "decreased use" [2324]. In this model, the microgravity conditions result in similar trends in skeletal muscle atrophy as seen in the unloading model [24]. Wronski and Morey-Horton described use of a cage to remove the weight-bearing function of rats by attaching their legs to their tails with a connector and mounting this to the top of the cage [25]. The rats are then only able to use their forelimbs to move around the cage.
During 3 to 28 days of unloading, electromyography of the soleus and gastrocnemius muscles showed initial decreases and then gradually returned to control level [26]. Soon after unloading, the total amount of protein per soleus muscle was an estimated 50% that of the control [27]. The decrease in protein was more dramatic in slow-twitch fibers than in fast-twitch fibers [827]. Myofibrillar protein constituted a large portion of the total protein loss [2829], and the losses were relatively greater in slow-twitch fibers. After 4 weeks of unloading, there was a 50% loss of myofibrillar protein in the soleus muscle, while no change in myofibrillar protein concentration was observed in the plantaris muscle [29]. The contractile tension and mass of both the soleus and plantaris muscles decreased by approximately 50%, and the soleus muscle showed changes in muscle contraction speed [29].
With regard to metabolic activity, there have been many reported changes in glycolytic and oxidative enzymes in the hindlimb unloading rodent model [30313233]. Lactate dehydrogenase activity was increased by 50% to 70% in single isolated fibers [3233], while dehydrogenase and phosphofructokinase activities were increased by 113% and 71%, respectively [32]. These changes occurred in slow-twitch fibers but not in fast-twitch fibers as little to no changes were observed in the fast-twitch glycolytic fibers of the gastrocnemius muscle [30].
Oxidative enzyme changes reflect the respiratory capacity of skeletal muscle. Previous studies have reported that the respiratory capacity of the entire soleus muscle was decreased during the unloading phase [3435]. However, single fibers of the soleus muscle showed 27% to 40% increases in succinate dehydrogenase activity [3637] and 69% increases in citrate synthase activity [34] during 1 to 4 weeks of hindlimb suspension. These observations can be attributed to the atrophic loss of skeletal muscle resulting in decreased fiber mass and thus decreased respiratory capacity.
Senescence of muscle severely affects its strength and size. In aging mice models, lean mass starts to decline after 18 months of age [38]. A review of grip strength data in C57BL/6 mice suggested that a pronounced decline in strength does not occur until 15 months of age but then significantly decreases between 15 and 28 months of age [3940].
Due to the fact that the specific tension of a muscle is closely related to the CSA of the fiber, muscle fiber size is regarded to be an important factor in strength. The contribution of the loss of muscle fiber and size to weakness in aged muscle has already been demonstrated by many studies with muscle biopsy of the vastus lateralis [540]. In these reports, aging resulted in an increase in the relative percentage of type I muscle fibers and the atrophic responses of the type II fibers [4041].
Throughout an organism's life span, there are losses in the total number and diameter of axonal fibers [42]. Older rats (28 and 30 months) showed a significant decrease in the number of medial gastrocnemius motor neurons compared to that in relatively younger rats (17 months) [6]. These findings suggest that there is a loss of conduction speed in motor neurons during aging [6].
However, there is conclusive evidence that even very old muscle can be strengthened through exercise therapy; thus, highlighting the importance of exercise in promoting healthy aging.
Protein turnover is the balance between protein synthesis and protein degradation. As myofibrils constitute 70% of the total protein within the muscle cell, protein turnover balance is very important in understanding atrophic responses. During decreased muscle use, there are alterations in protein turnover. A decreased rate of protein synthesis is a distinctive feature of various models of decreased use [434445]. After 15 days of immobilization, the rate of protein synthesis is stimulated by amino acid intake [46]. As a result, reduction in the basal synthesis rate of muscle protein is decreased, as is muscle mass.
Insulin-like growth factor 1-Akt-forkhead box O (IGF1-Akt-FoxO) is a major component of the signaling pathways that regulate the atrophic response [51]. Local injection of IGF-1 successfully inhibited atrophy caused by decreased use [52], and studies have shown that IGF-1 transgenic mice were protected from skeletal muscle atrophy during pathological conditions [5354]. The transgenic overexpression of Akt in a denervation mouse model showed resistance to skeletal muscle atrophy [5556]. Akt has a crucial role in the control of protein synthesis and degradation via the mammalian target of rapamycin and FoxO family, and the levels of Akt protein and its phosphorylation are remarkably decreased during hindlimb unloading [57].
Upregulation of the proteasome and autophagy-lysosome systems is common in atrophy conditions, with increased transcription of ubiquitin ligases such as atrogin-1 and cbl-b during bed rest [51]. This upregulation involves an increase in the transcription of atrogin-1, which promotes the degradation of MyoD, a key factor in activation of protein synthesis pathways [51]. During bed rest, there are small decreases in the mRNA transcription of α-actin and the β-myosin-heavy chain, and there is evidence that unloading downregulates both transcription and translation [45]. As a result, the synthesis rate dramatically decreased.
Aged muscle exhibits increased apoptosis in skeletal muscle cells [58]. Apoptosis is initiated via extrinsic stimuli and the downstream signaling pathways of tumor necrosis factor-α [59]. Apoptosis in aging skeletal muscle may involve mitochondrial-mediated signaling. Recent studies have shown a significant increase in the protein levels of procaspase-3 and caspase-3, the inactivated and activated forms of the enzyme, respectively [59]. The pro-apoptotic proteins apoptosis-inducing factor and Apaf-1 were also increased in the gastrocnemius muscle, suggesting that apoptotic potential increases with age [59]. Bax and caspase-9 activity also increased in the aged plantaris muscle, suggesting that there is a close link between aging-related apoptosis and the mitochondrial-meditated pathway.
The decreased use of skeletal muscle can result in decreased strength, decreased fiber size, and fiber type transformation (Table 1). In the aging model, there are distinctive atrophic responses that distinguish it from the other three models discussed. While the loss of skeletal muscle mass and function is a general outcome of decreased use, the main difference is the vulnerability of fiber types. In the aging model, the decrease in the function and size of skeletal muscle was mostly derived from the loss of type II muscle [8]. Thus, the muscles most affected by age-related atrophy were the type II fiber-dominant muscles such as the plantaris [8]. In the other three models discussed above, atrophy significantly affected muscles with type I fibers [4830]. These findings suggest that, during aging, the reduced muscle function is due to the loss of strength, while the muscle function loss in the other decreased use models might be due to changes in oxidative capacity caused by lack of movement [333435].
The animals in the immobilization, spinal cord transection, and hindlimb unloading models also showed skeletal muscle atrophy, but this was mostly observed in the soleus, a type I fiber-based skeletal muscle. Decreased oxidative capacity is observed during bed rest or immobilization [34], and there is a fiber transformation from type II to type I fibers [48]. These transformations and the loss of type I fibers are speculated to be due to the loss of weight-loading in the muscle of interest. Some studies have shown that gravity-sensitive genes drive certain types of myofiber heavy changes. The complete or partial loss of weight-loading might affect gene activation and result in changes in the composition and loss of specific muscle fibers.
Two general principles of muscle atrophy are that the atrophy of slow-twitch muscle fibers occurs before that of fast-twitch fiber, and that skeletal muscles in shortened positions have greater reductions in function. However, the atrophic changes in skeletal muscle that occur in different decreased use models are diverse. In addition, these models affect target skeletal muscle differently, with variable vulnerabilities and functional changes.
Due to the differences between the aging model and the other three models, the muscle of interest should be carefully considered. In early studies of skeletal muscle disuse, the immobilization and hindlimb unloading models were adopted to study the functional loss of skeletal muscle in aging [272829]. However, it is important to keep in mind that these two models have distinctive effects on the skeletal muscle system.
In conclusion, decreased use animal models are widely used to simulate different limitations in physical conditioning. The loss of physical activity always results in loss of skeletal mass and function. Given that different models have different physiological effects on skeletal muscle, care should be taken in the selection of an appropriate model for the research objectives and the muscle of interest.
Figures and Tables
Table 1
Comparison of Changes in the Soleus Muscle with Decreased Use Model
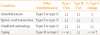
ACKNOWLEDGMENTS
This work was supported by the National Research Foundation of Korea funded by the Ministry of Science, ICT and Future Planning (Korea Mouse Phenotyping Project NRF-2013M3A9D 5072550, and MEST 2011-030135) and by the Ministry of Education (NRF-2014R1A1A2058645).
References
1. Ikemoto M, Nikawa T, Takeda S, Watanabe C, Kitano T, Baldwin KM, et al. Space shuttle flight (STS-90) enhances degradation of rat myosin heavy chain in association with activation of ubiquitin-proteasome pathway. FASEB J. 2001; 15:1279–1281.
2. Janssen I, Heymsfield SB, Wang ZM, Ross R. Skeletal muscle mass and distribution in 468 men and women aged 18-88 yr. J Appl Physiol (1985). 2000; 89:81–88.
3. Phillips SM, Glover EI, Rennie MJ. Alterations of protein turnover underlying disuse atrophy in human skeletal muscle. J Appl Physiol (1985). 2009; 107:645–654.
4. Jiang B, Roy RR, Edgerton VR. Expression of a fast fiber enzyme profile in the cat soleus after spinalization. Muscle Nerve. 1990; 13:1037–1049.
5. Nilwik R, Snijders T, Leenders M, Groen BB, van Kranenburg J, Verdijk LB, et al. The decline in skeletal muscle mass with aging is mainly attributed to a reduction in type II muscle fiber size. Exp Gerontol. 2013; 48:492–498.
6. Kido A, Tanaka N, Stein RB. Spinal excitation and inhibition decrease as humans age. Can J Physiol Pharmacol. 2004; 82:238–248.
7. Ohira Y, Yoshinaga T, Ohara M, Kawano F, Wang XD, Higo Y, et al. The role of neural and mechanical influences in maintaining normal fast and slow muscle properties. Cells Tissues Organs. 2006; 182(3-4):129–142.
8. Thomason DB, Booth FW. Atrophy of the soleus muscle by hindlimb unweighting. J Appl Physiol (1985). 1990; 68:1–12.
9. Caron AZ, Drouin G, Desrosiers J, Trensz F, Grenier G. A novel hindlimb immobilization procedure for studying skeletal muscle atrophy and recovery in mouse. J Appl Physiol (1985). 2009; 106:2049–2059.
10. Jokl P, Konstadt S. The effect of limb immobilization on muscle function and protein composition. Clin Orthop Relat Res. 1983; (174):222–229.
11. Herbert RD, Balnave RJ. The effect of position of immobilisation on resting length, resting stiffness, and weight of the soleus muscle of the rabbit. J Orthop Res. 1993; 11:358–366.
12. Roy RR, Baldwin KM, Edgerton VR. The plasticity of skeletal muscle: effects of neuromuscular activity. Exerc Sport Sci Rev. 1991; 19:269–312.
13. Adams GR, Caiozzo VJ, Baldwin KM. Skeletal muscle unweighting: spaceflight and ground-based models. J Appl Physiol (1985). 2003; 95:2185–2201.
14. Jozsa L, Thoring J, Jarvinen M, Kannus P, Lehto M, Kvist M. Quantitative alterations in intramuscular connective tissue following immobilization: an experimental study in the rat calf muscles. Exp Mol Pathol. 1988; 49:267–278.
15. Booth FW, Kelso JR. Effect of hind-limb immobilization on contractile and histochemical properties of skeletal muscle. Pflugers Arch. 1973; 342:231–238.
16. Spector SA, Simard CP, Fournier M, Sternlicht E, Edgerton VR. Architectural alterations of rat hind-limb skeletal muscles immobilized at different lengths. Exp Neurol. 1982; 76:94–110.
17. Edgerton VR, Barnard RJ, Peter JB, Maier PA, Simpson DR. Properties of immobilized hind-limb muscles of the Galago senegalensis. Exp Neurol. 1975; 46:115–131.
18. Booth FW. Effect of limb immobilization on skeletal muscle. J Appl Physiol Respir Environ Exerc Physiol. 1982; 52:1113–1118.
19. Andersen JL, Mohr T, Biering-Sorensen F, Galbo H, Kjaer M. Myosin heavy chain isoform transformation in single fibres from m. vastus lateralis in spinal cord injured individuals: effects of long-term functional electrical stimulation (FES). Pflugers Arch. 1996; 431:513–518.
20. Talmadge RJ, Roy RR, Jiang B, Edgerton VR. Myofibrillar ATPase activity of feline muscle fibers expressing slow and fast myosin heavy chains. J Histochem Cytochem. 1995; 43:811–819.
21. Martin TP, Stein RB, Hoeppner PH, Reid DC. Influence of electrical stimulation on the morphological and metabolic properties of paralyzed muscle. J Appl Physiol (1985). 1992; 72:1401–1406.
22. Shields RK. Muscular, skeletal, and neural adaptations following spinal cord injury. J Orthop Sports Phys Ther. 2002; 32:65–74.
23. Morey-Holton ER, Globus RK. Hindlimb unloading rodent model: technical aspects. J Appl Physiol (1985). 2002; 92:1367–1377.
24. Lawler JM, Song W, Demaree SR. Hindlimb unloading increases oxidative stress and disrupts antioxidant capacity in skeletal muscle. Free Radic Biol Med. 2003; 35:9–16.
25. Wronski TJ, Morey-Holton ER. Skeletal response to simulated weightlessness: a comparison of suspension techniques. Aviat Space Environ Med. 1987; 58:63–68.
26. Alford EK, Roy RR, Hodgson JA, Edgerton VR. Electromyography of rat soleus, medial gastrocnemius, and tibialis anterior during hind limb suspension. Exp Neurol. 1987; 96:635–649.
27. Goldspink DF, Morton AJ, Loughna P, Goldspink G. The effect of hypokinesia and hypodynamia on protein turnover and the growth of four skeletal muscles of the rat. Pflugers Arch. 1986; 407:333–340.
28. Jaspers SR, Fagan JM, Tischler ME. Biochemical response to chronic shortening in unloaded soleus muscles. J Appl Physiol (1985). 1985; 59:1159–1163.
29. Tsika RW, Herrick RE, Baldwin KM. Effect of anabolic steroids on skeletal muscle mass during hindlimb suspension. J Appl Physiol (1985). 1987; 63:2122–2127.
30. Bigard AX, Boehm E, Veksler V, Mateo P, Anflous K, Ventura-Clapier R. Muscle unloading induces slow to fast transitions in myofibrillar but not mitochondrial properties. Relevance to skeletal muscle abnormalities in heart failure. J Mol Cell Cardiol. 1998; 30:2391–2401.
31. Grichko VP, Heywood-Cooksey A, Kidd KR, Fitts RH. Substrate profile in rat soleus muscle fibers after hindlimb unloading and fatigue. J Appl Physiol (1985). 2000; 88:473–478.
32. Fitts RH, Brimmer CJ, Heywood-Cooksey A, Timmerman RJ. Single muscle fiber enzyme shifts with hindlimb suspension and immobilization. Am J Physiol. 1989; 256(5 Pt 1):C1082–C1091.
33. Hauschka EO, Roy RR, Edgerton VR. Size and metabolic properties of single muscle fibers in rat soleus after hindlimb suspension. J Appl Physiol (1985). 1987; 62:2338–2347.
34. Fell RD, Steffen JM, Musacchia XJ. Effect of hypokinesia-hypodynamia on rat muscle oxidative capacity and glucose uptake. Am J Physiol. 1985; 249(3 Pt 2):R308–R312.
35. Simard C, Lacaille M, Vallieres J. Enzymatic adaptations to suspension hypokinesia in skeletal muscle of young and old rats. Mech Ageing Dev. 1985; 33:1–9.
36. Graham SC, Roy RR, West SP, Thomason D, Baldwin KM. Exercise effects on the size and metabolic properties of soleus fibers in hindlimb-suspended rats. Aviat Space Environ Med. 1989; 60:226–234.
37. Hauschka EO, Roy RR, Edgerton VR. Periodic weight support effects on rat soleus fibers after hindlimb suspension. J Appl Physiol (1985). 1988; 65:1231–1237.
38. Hamrick MW, Ding KH, Pennington C, Chao YJ, Wu YD, Howard B, et al. Age-related loss of muscle mass and bone strength in mice is associated with a decline in physical activity and serum leptin. Bone. 2006; 39:845–853.
39. Fahlstrom A, Yu Q, Ulfhake B. Behavioral changes in aging female C57BL/6 mice. Neurobiol Aging. 2011; 32:1868–1880.
40. Kallman DA, Plato CC, Tobin JD. The role of muscle loss in the age-related decline of grip strength: cross-sectional and longitudinal perspectives. J Gerontol. 1990; 45:M82–M88.
41. Kortebein P, Ferrando A, Lombeida J, Wolfe R, Evans WJ. Effect of 10 days of bed rest on skeletal muscle in healthy older adults. JAMA. 2007; 297:1772–1774.
42. Kawamura Y, O'Brien P, Okazaki H, Dyck PJ. Lumbar motoneurons of man II: the number and diameter distribution of large- and intermediate-diameter cytons in "motoneuron columns" of spinal cord of man. J Neuropathol Exp Neurol. 1977; 36:861–870.
43. de Boer MD, Selby A, Atherton P, Smith K, Seynnes OR, Maganaris CN, et al. The temporal responses of protein synthesis, gene expression and cell signalling in human quadriceps muscle and patellar tendon to disuse. J Physiol. 2007; 585(Pt 1):241–251.
44. Gibson JN, Smith K, Rennie MJ. Prevention of disuse muscle atrophy by means of electrical stimulation: maintenance of protein synthesis. Lancet. 1988; 2:767–770.
45. Glover EI, Phillips SM, Oates BR, Tang JE, Tarnopolsky MA, Selby A, et al. Immobilization induces anabolic resistance in human myofibrillar protein synthesis with low and high dose amino acid infusion. J Physiol. 2008; 586(Pt 24):6049–6061.
46. Bohe J, Low A, Wolfe RR, Rennie MJ. Human muscle protein synthesis is modulated by extracellular, not intramuscular amino acid availability: a dose-response study. J Physiol. 2003; 552(Pt 1):315–324.
47. Jagoe RT, Goldberg AL. What do we really know about the ubiquitin-proteasome pathway in muscle atrophy? Curr Opin Clin Nutr Metab Care. 2001; 4:183–190.
48. Taillandier D, Aurousseau E, Meynial-Denis D, Bechet D, Ferrara M, Cottin P, et al. Coordinate activation of lysosomal, Ca2+-activated and ATP-ubiquitin-dependent proteinases in the unweighted rat soleus muscle. Biochem J. 1996; 316(Pt 1):65–72.
49. Tawa NE Jr, Odessey R, Goldberg AL. Inhibitors of the proteasome reduce the accelerated proteolysis in atrophying rat skeletal muscles. J Clin Invest. 1997; 100:197–203.
50. Ogawa T, Furochi H, Mameoka M, Hirasaka K, Onishi Y, Suzue N, et al. Ubiquitin ligase gene expression in healthy volunteers with 20-day bedrest. Muscle Nerve. 2006; 34:463–469.
51. Bonaldo P, Sandri M. Cellular and molecular mechanisms of muscle atrophy. Dis Model Mech. 2013; 6:25–39.
52. Stitt TN, Drujan D, Clarke BA, Panaro F, Timofeyva Y, Kline WO, et al. The IGF-1/PI3K/Akt pathway prevents expression of muscle atrophy-induced ubiquitin ligases by inhibiting FOXO transcription factors. Mol Cell. 2004; 14:395–403.
53. Schulze PC, Fang J, Kassik KA, Gannon J, Cupesi M, MacGillivray C, et al. Transgenic overexpression of locally acting insulin-like growth factor-1 inhibits ubiquitin-mediated muscle atrophy in chronic left-ventricular dysfunction. Circ Res. 2005; 97:418–426.
54. Song YH, Li Y, Du J, Mitch WE, Rosenthal N, Delafontaine P. Muscle-specific expression of IGF-1 blocks angiotensin II-induced skeletal muscle wasting. J Clin Invest. 2005; 115:451–458.
55. Lai KM, Gonzalez M, Poueymirou WT, Kline WO, Na E, Zlotchenko E, et al. Conditional activation of akt in adult skeletal muscle induces rapid hypertrophy. Mol Cell Biol. 2004; 24:9295–9304.
56. Mammucari C, Milan G, Romanello V, Masiero E, Rudolf R, Del Piccolo P, et al. FoxO3 controls autophagy in skeletal muscle in vivo. Cell Metab. 2007; 6:458–471.
57. Stevenson EJ, Giresi PG, Koncarevic A, Kandarian SC. Global analysis of gene expression patterns during disuse atrophy in rat skeletal muscle. J Physiol. 2003; 551(Pt 1):33–48.
58. Wohlgemuth SE, Seo AY, Marzetti E, Lees HA, Leeuwenburgh C. Skeletal muscle autophagy and apoptosis during aging: effects of calorie restriction and life-long exercise. Exp Gerontol. 2010; 45:138–148.
59. Dupont-Versteegden EE. Apoptosis in muscle atrophy: relevance to sarcopenia. Exp Gerontol. 2005; 40:473–481.