Abstract
Background
It has been recognized that a defect in klotho gene expression accelerates the degeneration of multiple age-sensitive traits. Accumulating evidence indicates that aging is associated with declines in cognitive function and the activity of growth hormone (GH)/insulin-like growth factor-1 (IGF-1).
Methods
In this study, we examined whether a GH-releaser diet could be effective in protecting against cognitive impairment in klotho mutant mice.
Results
The GH-releaser diet significantly induced the expression of IGF-1 and IGF-1 receptors in the hippocampus of klotho mutant mice. Klotho mutant mice showed significant memory impairments as compared with wild-type mice. In addition, the klotho mutation significantly decreased the expression of cell survival/antiapoptotic factors, including phospho-Akt (p-Akt)/phospho-glycogen synthase kinase3β (p-GSK3β), phospho-extracellular signal-related kinase (p-ERK), and Bcl-2, but significantly increased those of cell death/proapoptotic factors, such as phospho-c-jun N-terminal kinase (p-JNK), Bax, and cleaved caspase-3 in the hippocampus. Treatment with GH-releaser diet significantly attenuated both decreases in the expression of cell survival/antiapoptotic factors and increases in the expression of cell death/proapoptotic factors in the hippocampus of klotho mutant mice. In addition, klotho mutation-induced oxidative stress was significantly attenuated by the GH-releaser diet. Consequently, a GH-releaser diet significantly improved memory function in the klotho mutant mice. GH-releaser diet-mediated actions were significantly reversed by JB-1, an IGF-1 receptor antagonist.
The klotho gene was named after the Greek goddess who purportedly spun the thread of life; this gene has been shown to encode a type I membrane protein with an extracellular domain [1,2,3]. The klotho gene is mainly expressed in tissues that function in the regulation of calcium and phosphate homeostasis, including the distal convoluted tubules in the kidney, as well as parathyroid hormone-secreting cells and the epithelium of the choroid plexus of the brain [3,4]. The brain, pituitary gland, skeletal muscle, and many other peripheral organs also show detectable levels of klotho gene expression [3]. Klotho proteins have been predicted to be localized on the cell surface of klotho-expressing cells because the klotho gene encodes a type 1 membrane protein. However, a large amount of klotho protein is detectable in the cytoplasm; its intracellular distribution mostly overlaps with the endoplasmic reticulum and Golgi apparatus [4]. The extracellular domain of klotho, as a coreceptor for fibroblast growth factor, plays an important role in maintaining phosphate and calcium homeostasis [5]. The secreted form of klotho, which is produced by shedding of the extracellular domain of klotho, is secreted into the blood and cerebrospinal fluid and shows various physiological effects, including the modulation of growth factor signaling [3,6].
Klotho mutant mice, which are defective in klotho gene expression, develop multiple age-related syndromes even at the age of 4 to 5 weeks, including growth retardation, hypogonadotropic hypogonadism, rapid thymic involution, skin atrophy, sarcopenia, vascular calcification, osteopenia, pulmonary emphysema, cognition impairment, hearing disturbance, and motor neuron degeneration; they typically die prematurely around 2 months of age [6]. In contrast, the introduction of a normal klotho gene into these mutant mice improves their phenotypes [4], and overexpression of this gene in normal wild-type mice significantly extends their lifespans [7]. Thus, the klotho gene may function as an age suppressor gene that extends the lifespan when overexpressed and accelerates aging when disrupted [8].
Although klotho mutant mice are considered to be a novel animal model for accelerated human aging, these mice do not reveal some phenotypes usually seen in aged humans, such as brain atrophy with the deposition of amyloid or senile plaques [4,9,10]. In previous studies, however, we reported cognitive impairment in klotho mutant mice [9,11]. We showed that the antideath gene/protein, Bcl-2 or Bcl-xL, was downregulated while the prodeath molecule, Bax, was upregulated in the hippocampus of these mutant mice [9]. Our recent study has suggested that inactivation of the janus kinase 2/signal transducer and activator of transcription 3 signaling axis and M1 muscarinic cholinergic receptor downregulation play a critical role in cognitive impairment in klotho mutant mice [11]. Immunocytochemically, Li et al. [12] indicated that synaptic structures and synaptophysin were reduced in number and expression, respectively, in the CA3 region of these animals. These results have suggested that the klotho gene may be essential for maintaining cognitive function in aging organisms.
The activities of growth hormone (GH) and insulin-like growth factor-1 (IGF-1) decline with aging [13], which could contribute to age-related cognitive performance decay [14]. Although GH might exert a positive effect in preventing the development of Alzheimer disease (AD), exogenous GH treatment in healthy elderly participants or AD patients is not an attractive tool due to its potentially harmful side effects, injection requirements and high cost [15]. Thus, methods for increasing endogenous GH secretion and subsequent IGF-1 synthesis by oral intake might be a better alternative. As the oral administration of amino acids (i.e., arginine, glutamine, glycine, and lysine) has been found to increase the release of endogenous GH [16], supplementation with these amino acids might be a beneficial pharmacological intervention. We previously demonstrated that a "GH-releaser diet" significantly attenuated β-amyloid (Aβ) (1-42)-induced memory impairment [17] via stimulation of the hippocampal IGF-1 receptor [18].
Although previous reports suggest that klotho may have an inhibitory effect on insulin/IGF-1 activity, the mechanism by which klotho modulates the activity of insulin/IGF-1 receptors in cognitive function remains unknown. Therefore, we examined whether the GH-releaser diet could effectively protect against cognitive impairment in klotho mutant mice. In this study, the GH-releaser diet significantly attenuated memory deficits, and these effects were significantly counteracted by treatment with GH or IGF-1 receptor antagonists.
All animals were treated in accordance with the National Institutes of Health (NIH) Guide for the Humane Care and Use of Laboratory Animals (NIH Publication No. 85 23, 1985; www.dels.nas.edu/ila). The present study was performed in accordance with the Institute for Laboratory Research guidelines for the care and use of laboratory animals. Mice were maintained under a 12-hour light/12-hour dark cycle and fed ad libitum. Since klotho mutant mice are infertile, wild-type and klotho mutant mice were generated by crossing heterozygous klotho mutant mice [4,9,11]. Prior to weaning, tail specimens were collected from each animal, and DNA was extracted to genotype wild-type- and klotho mutant mice by polymerase chain reaction (PCR) using primer pairs specific for each genotype; 5'-TTGTGGAGATTGGAAGTGGACGAAAGAG-3' (forward), 5'-CGCCCCGACCGGAGCTGAGAGTA-3' (klotho mutant reverse), 5'-CTGGACCCCCTGAAGCTGGAGTTAC-3' (wild-type reverse). The products were amplified in a GeneAmp PCR System 9700 (Applied Biosystems, Foster City, CA, USA) using the following PCR parameters: an initial denaturation at 94℃ for 5 minutes, 30 cycles of 94℃ for 30 seconds, 65℃ for 30 seconds, 72℃ for 30 seconds, and 72℃ for 5 minutes, followed by electrophoresis on 1% agarose gels with ethidium bromide and photography under ultraviolet (UV) light. Six-week-old wild-type or klotho mutant mice were used in the present study.
Forty-day-old mice were anesthetized with pentobarbital (40 mg/kg, intraperitoneally) and placed in a stereotaxic apparatus (David Kopf Instruments, Tujunga, CA, USA). A stainless steel guide cannula (AG-4, Eicom, Kyoto, Japan) was implanted into the right lateral ventricle (stereotaxic coordinates: 0.5 mm posterior to the bregma, 1 mm right to the midline, and 2 mm ventral to the dura) [11,18]. Microinfusion into the lateral ventricle was performed through a microinfusion cannula (AMI-4, Eicom) at a rate of 1 µL/min using a microinjection pump (CMA/100, CMA, Solna, Sweden). The microinfusion cannula was kept in place for 1 minute after infusion to avoid backflow. Guide cannula implantation did not affect the behavior of the subjects. Subsequent to guide cannula implantation, each animal was housed in a single cage in order to safely maintain the integrity of the implantation during the experimental period.
Drug treatment started after 24 hours of recovery from the guide cannula implantation. Mice received a GH-releaser diet [17,18], which is composed of 5% GH-releaser (a mixture of l-arginine/l glutamine/l-lysine/glycine at a ratio of 37:30: 18.5:14.5) and 95% vehicle diet (45% sucrose, 20% casein, 15% cornstarch, 7% corn oil, 3% cellulose, 3% mineral mixture, 1.5% vitamin mixture, 0.3% dl-methionine, and 0.2% choline bitartrate), or a vehicle diet during the entire experimental period (25 days). JB-1 (IGF-1 receptor antagonist, Bachem Bioscience, Inc., King of Prussia, PA, USA) was dissolved in artificial cerebrospinal fluid (aCSF; 150 mM NaCl, 1.8 mM CaCl2, 1.2 mM MgSO4, 2 mM K2HPO4, 10 mM glucose, pH 7.4) and infused (1 µg/5 µL/5 min, once daily for 25 days) into the lateral ventricle. The dose of JB-1 used in this study was selected based on previous studies [18]. Twenty days after beginning the GH-releaser diet, novel object recognition test and conditioned fear learning test were performed. Mice were sacrificed for Western blot, reverse transcription-PCR (RT-PCR) or neurochemical analyses at 15 minutes after the test trial of the conditioned fear learning test (Fig. 1).
The novel object recognition test was performed as described previously with a slight modification [9,11]. During the training session, two different objects were floor-fixed in a symmetric position from the center of the open field box (40×40×35 high cm), 20 cm from each and 10 cm from the nearest wall, and each mouse was allowed to explore the objects for 10 minutes. Twenty-four hours after the training session, mice were subjected to the retention session. One of the familiar objects used during training was replaced by a novel object, and then each mouse was allowed to explore the objects for 10 minutes. Time spent exploring each object was recorded and analyzed with a video tracking system (EthoVision, Noldus, the Netherlands). Novel object recognition was expressed as an "exploration of a novel object (%)," the percentage of time spent exploring the novel object over the total time spent exploring both objects.
Mice were placed in a neutral cage (30×30×35 high in cm), and the freezing response was measured for 1 minute in the absence of sound (preconditioning phase). In the conditioning phase, each mouse was placed in a training cage (25×30×11 high in cm) equipped with a metal floor, and the mice were allowed to explore the cage freely for 2 minutes, and then a 15-second tone (85 dB) was delivered (conditioned stimulus). During the last 5 seconds of the tone stimulus, a foot shock of 0.8 mA was delivered as an unconditioned stimulus though a shock generator. This procedure was repeated four times at 15-second intervals. Twenty-four hours after the conditioning, context-dependent tests were carried out. Mice were placed in the training cage, and the freezing response was measured for 2 minutes in the absence of the conditioned stimulus [9,11].
Tissues were homogenized in a lysis buffer, containing 200 mM Tris HCl (pH 6.8), 1% SDS, 5 mM ethyleneglycol tetraacetic acid, 5 mM ethylenediaminetetraacetic acid, 10% glycerol, 1×phosphatase inhibitor cocktail I, 1×protease inhibitor cocktail. The supernatant fraction was subsequently centrifuged at 30,000 g for 30 minutes. Proteins (20 to 50 µg/lane) were separated by 10% to 12% sodium dodecyl sulfate-polyacrylamide gel electrophoresis and transferred onto polyvinylidene difluoride membranes. Following transfer, membranes were preincubated with 5% nonfat milk and incubated overnight at 4℃ with anti-Akt (1:5,000, Cell Signaling Technology, Inc., Danvers, MA, USA), antiphospho-Akt (anti-p-Akt) at Thr308 (1:1,000, Cell Signaling Technology, Inc.), antiglycogen synthase kinase3β (anti-GSK3β; 1:3,000, BD Biosciences, San Jose, CA, USA), antiphospho-GSK3β at Ser9 (anti-p-GSK3β; 1:750, Cell Signaling Technology, Inc.), anticalcium/calmodulin-dependent kinase II (anti-CaMKII; 1:1,000, Millipore, Bedford, MA, USA), anti-phospho-CaMKII (anti-p-CaMKII; 1:1,000, Millipore), antiextracellular signal-related kinase (anti-ERK; 1:5,000, Cell Signaling Technology, Inc.), anti-phospho-ERK (anti-p-ERK; 1:1,000, Cell Signaling Technology, Inc.), anti-c-jun N-terminal kinase (anti-JNK; 1:2,000, Cell Signaling Technology, Inc.), anti-phosphor-JNK (anti-p-JNK; 1:1,000, Cell Signaling Technology, Inc.), anti-p38 (1:1,000, Cell signaling Technology, Inc.), anti-phospho-p38 (anti-p-p38; 1:500, Cell Signaling Technology, Inc.), anti-cAMP responsive element binding protein (anti-CREB; 1:1,000, Cell Signaling Technology, Inc.), anti-phospho-CREB (anti-p-CREB; 1:500, Cell Signaling Technology, Inc.), Bcl-2 (1:500; Santa Cruz Biotechnology, Inc., Santa Cruz, CA, USA), Bcl-xL (1:1,000, Cell Signaling Technology, Inc.), Bax (1:500, Santa Cruz Biotechnology, Inc.), or cleaved caspase-3 (1:1,000, Cell Signaling Technology, Inc.). After incubation with primary antibody, membranes were incubated with horseradish peroxidase-conjugated secondary antirabbit immunoglobulin G (IgG; 1:1,000, GE Healthcare, Piscataway, NJ, USA), antimouse IgG (1:1,000, Sigma, St. Louis, MO, USA) or antigoat IgG (1:1,000, Sigma) for 2 hours. Subsequent visualization was performed using the ECL plus enhanced chemiluminescence system (GE Healthcare). Relative band intensities were quantified using PhotoCapt MW version 10.01 (Vilber Lourmat, Marne la Vallée, France), and each intensity was then normalized to the β-actin intensity.
Expression of IGF-1 and IGF-1 receptors was assessed using semi-quantitative RT-PCR to analyze mRNA levels. Total RNA was isolated from hippocampal tissues using an RNeasy Mini Kit (Qiagen, Valencia, CA, USA) according to the manufacturer's instructions. Reverse transcription reactions were carried out using avian myeloblastosis virus reverse transcriptase (Promega, Madison, WI, USA) and random oligonucleotide primers for 1 hour at 37℃. PCR amplification was performed using 35 cycles of denaturation at 94℃ for 1 minute, annealing at 60℃ for 2 minutes and extension at 72℃ for 1 minute. The following primer sequences were used: IGF-1 specific primers [18], forward: 5'-GGA CCA GAG ACC CTT TGC GGG G-3' and reverse: 5'-GGC TGC TTT TGT AGG CTT CAG TGG-3'; IGF 1 receptor specific primers [19], forward: 5'-GAC ATC CGC AAC GAC TAT CAG-3' and reverse: 5'-GTA GTT ATT GGA CAC CGC ATC-3'; glyceraldehyde-3-phosphate dehydrogenase specific primers [13], forward: 5' ACC-ACA-GTC-CAT-GCC-ATC-AC-3' and reverse: 5'-TCC-ACC-ACC-CTG-TTG-CTG-TA-3'. PCR products were separated on 2% agarose gels containing ethidium bromide and visualized under UV light. The quantitative analysis of mRNA was performed using PhotoCapt MW version 10.01.
Hippocampal tissues were homogenized in phosphate buffered saline (PBS) to measure malondialdehyde (MDA), protein carbonyl and reactive oxygen species. The amount of lipid peroxidation was determined by measuring the accumulation of thiobarbituric acid-reactive substances in hippocampal homogenates and is expressed as MDA content. In brief, 0.1 mL of the homogenate (or standard solutions prepared daily from 1,1,3,3-tetra-methoxypropane) and 0.75 mL of the working solution (thiobarbituric acid 0.37% and perchloric acid 6.4%, 2:1 v/v) were mixed and heated to 95℃ for 1 hour. After cooling (10 minutes in an ice water bath), the flocculent precipitate was removed by centrifugation at 3,200 ×g for 10 minutes. The supernatant was neutralized and filtered prior to injection on an octadecylsilyl 5 µm column. The mobile phase consisted of 50 mM PBS (pH 6.0):methanol (58:42, v/v). Isocratic separation was performed with 1.0 mL/min flow rate and detection at 532 nm using a UV/VIS HPLC-Detector (Model 486, Waters Associates, Milford, MA, USA) [20].
The extent of protein oxidation was assessed by measuring the content of protein carbonyl groups, which was determined spectrophotometrically with the 2,4-dinitrophenylhydrazine (DNPH)-labeling procedure [20] as described by Oliver et al. [19]. The results are expressed as nmoL of DNPH incorporated/mg protein based on the extinction coefficient for aliphatic hydrazones of 21 mM-1 cm-1. Protein was measured using the BCA protein assay reagent (Pierce, Rockford, IL, USA).
Data were analyzed using the SPSS version 19.0 (IBM Co., Armonk, NY, USA). Three-way analysis of variance was performed for the effect of the klotho gene mutation, the GH-releaser diet and JB-1. Post hoc multiple pair-wise comparisons with Bonferroni's correction were then followed. P values of <0.05 were considered statistically significant.
As shown in Fig. 2, klotho mutant mice treated with aCSF and vehicle diet showed significantly reduced hippocampal IGF-1 and IGF-1 receptor mRNA expression, compared to wild-type mice (P<0.01). These decrements in the levels of IGF-1 and IGF-1 receptor mRNA were significantly blocked by the GH-releaser diet (IGF-1, P<0.01; IGF-1 receptor, P<0.05). In contrast, the GH-releaser diet did not affect hippocampal IGF-1 and IGF-1 receptor mRNA expression in wild-type mice. JB-1, an IGF-1 receptor antagonist, significantly blocked GH-releaser diet-mediated increases in IGF-1 and IGF-1 receptor mRNA expression in klotho mutant mice (IGF-1, P<0.01; IGF-1 receptor, P<0.05).
Total Akt was not changed in klotho mutant mice treated with aCSF and a vehicle diet, while the p-Akt/Akt ratio was significantly decreased compared to wild-type mice (P<0.01) (Fig. 3A). The GH-releaser diet significantly attenuated (P<0.01) a decrease in hippocampal p-Akt levels in the klotho mutant mice. A decrease in the ratio of p-GSK3β/GSK3β in klotho mutant mice (P<0.01) (Fig. 3B) was also significantly increased after the GH-releaser diet (P<0.01). JB-1 significantly blocked these actions on the hippocampal ratio of p-Akt/Akt (P<0.01) and p-GSK3β/GSK3β (P<0.05) in klotho mutant mice (Fig. 3A, B). A GH-releaser diet did not affect the expression of CaMKII and p-CaMKII in wild-type or klotho mutant mice (Fig. 3C).
The ratio of p-ERK/ERK and p-CREB/CREB was significantly decreased in klotho mutant mice (P<0.01) (Fig. 4A, D). The GH releaser diet significantly increased the hippocampal ratio of p-ERK/ERK (P<0.01) and p-CREB/CREB (P<0.05) in klotho mutant mice, which was significantly reversed by JB-1 (p-ERK/ERK, P<0.01; p-CREB/CREB, P<0.05). On the other hand, the increase in the ratio of p-JNK/JNK in klotho mutant mice (P<0.01) was significantly decreased after the GH-releaser diet (P<0.01) (Fig. 4B). JB-1 significantly blocked these actions in klotho mutant mice (P<0.05). However, the GH-releaser diet did not affect the expression and phosphorylation of p38 (Fig. 4C).
The levels of Bax and cleaved caspase-3 were significantly increased in the hippocampus of klotho mutant mice, whereas Bcl-2 and Bcl-xL were substantially decreased (P<0.01) (Fig. 5). Increases in the expression of Bax and cleaved caspase-3 were significantly reduced by the GH-releaser diet (P<0.01), which was reversed by JB-1 (Bax, P<0.01; cleaved caspase-3, P<0.05) (Fig. 5A, B). Decreases in the expression of Bcl-2 and Bcl-xL were significantly recovered in the presence of the GH-releaser diet in klotho mutant mice (P<0.01) (Fig. 5C, D). JB-1 significantly blocked these actions of the GH-releaser diet in klotho mutant mice (Bcl-2, P<0.01; Bcl-xL, P<0.05).
To determine the effect of the GH-releaser diet on oxidative stress, we examined lipid peroxidation (MDA) and protein oxidation (protein carbonyl) in the hippocampus of klotho mutant mice. The levels of MDA and protein carbonyl were significantly increased in klotho mutant mice (MDA, P<0.05; protein carbonyl, P<0.01). The GH-releaser diet significantly reduced the levels of MDA and protein carbonyl (MDA, P<0.05; protein carbonyl, P<0.01), and these effects were significantly reversed by JB-1 (protein carbonyl, P<0.05) (Fig. 6).
In the training trial of the novel object recognition test, wild-type and klotho mutant mice did not show any exploratory preference between objects (data not shown). In the test trial, the exploratory preference for the novel object, as expressed in the recognition index, was significantly decreased in klotho mutant mice compared with wild-type mice (P<0.01). The GH-releaser diet significantly increased the recognition index in klotho mutant mice (P<0.01), and this cognitive enhancing effect of the GH-releaser was significantly inhibited by JB-1 (P<0.05) (Fig. 7A).
During the preconditioning trial of the context-dependent conditioned fear learning test, mice showed little freezing behavior, and no statistical difference was observed among groups. In the retention trials (24 hours after the conditioning trial), klotho mutant mice showed significantly less freezing response compared with wild-type mice (P<0.01). The GH-releaser diet significantly enhanced the freezing response in klotho mutant mice (P<0.01). This enhancement was significantly blocked by JB-1 (P<0.05) (Fig. 7B). However, the GH-releaser diet affected none of these memory tests in the wild-type mice (Fig. 7).
The present study demonstrated that the GH-releaser diet significantly attenuated memory deficits in klotho mutant mice. The GH-releaser diet was shown to affect hippocampal changes in IGF-1/IGF-1-receptor mRNA expression, phosphorylation of Akt/GSK3β and ERK/CREB and the levels of apoptosis-related factors. In addition, the GH-releaser diet significantly decreased oxidative stress, such as lipid peroxidation and protein oxidation. These effects of the GH-releaser diet were significantly counteracted by treatment with JB-1, an IGF-1 receptor antagonist, suggesting that GH-releaser diet-mediated cognitive enhancement is stimulated via the IGF-1 receptor in klotho mutant mice.
The ability of klotho to inhibit insulin/IGF-1 receptors may contribute to its antiaging properties since partial inhibition of an insulin-like signaling pathway was reported as one of the evolutionarily conserved mechanisms for suppressing aging. Klotho-deficient mice are hypoglycemic, hypoinsulinemic and extremely sensitive to insulin [21], whereas klotho-overexpressing transgenic mice are resistant to insulin and IGF-1 [7]. Secreted klotho had enough activity to inhibit insulin/IGF-1-induced autophosphorylation of the insulin/IGF-1 receptor [7,22] and conferred oxidative stress resistance via activation of FoxO transcription factors that are negatively regulated by insulin/IGF-1 signaling [23]. Klotho's effects on insulin signaling or oxidative stress resistance may occur either via klotho expression in tissues involved in carbohydrate metabolism or as a hormone [24]. Therefore, it has become increasingly clear that partial or tissue-specific inhibition of the insulin-like signaling pathway is a mechanism for antiaging and life span extension. However, the mechanism by which secreted klotho inhibits activity of insulin/IGF-1 receptors in the brain remains to be determined.
Recently, Lorenzi and colleagues [25] demonstrated that the soluble klotho protein does not inhibit IGF-1 and/or insulin signaling, arguing against a direct role of klotho in insulin signaling. Conditioned media from cells expressing the klotho protein failed to inhibit IGF-1 signaling or insulin signaling in HEK cells, rat L6 or human HepG2 cells, although the klotho protein in conditioned media is bioactive [25]. Their results stand in contrast with the data published by Kurosu et al. [7], who showed potent inhibition of insulin and IGF-1 signaling by purified murine recombinant klotho protein in L6 cells. Those discrepancies may be due to the inactivation of klotho bioactivity during the purification procedure or to species restrictions. These findings that native, bioactive klotho protein fail to inhibit insulin signaling raises the possibility that the GH-releaser diet might attenuate cognitive impairment in klotho mutant mice via activation of IGF-1 signaling.
In the present study, the GH-releaser diet significantly attenuated memory deficits and reduced oxidative stress in klotho mutant mice. These results correlated with previously reported protective effects of a GH-releaser diet on Aβ (1-42)-induced learning impairment [17,18]. Given that the GH-releaser diet showed no significant changes in wild-type mice, we suggest that it can act more effectively to protect from various neuronal damages. To address the possibility that the neuroprotective effects reported in the present study were mediated by an IGF-1 pathway, we examined mRNA levels of IGF-1 and IGF-1 receptors in the hippocampus from klotho mutant mice. We detected significant increases in IGF-1 and IGF-1 receptors by the GH-releaser diet and further confirmed these effects of the GH-releaser diet using an IGF-1 receptor antagonist. The GH-releaser diet was also shown to decrease the levels of lipid peroxidation and protein oxidation, and treatment with IGF-1 receptor antagonist aggravated memory deficits in klotho mutant mice. These results confirmed that the IGF-1 pathway might be involved in attenuating memory impairment in klotho mutant mice by reducing oxidative stress.
Furthermore, the present study indicated that the GH-releaser diet activated both the PI3K/Akt and the ERK pathways in the hippocampus of klotho mutant mice, which is in agreement with the study by Frago et al. [26]. Synthetic GH secretagogues (GHS) and the endogenous ligand ghrelin have been found to have cardioprotective properties in several in vivo studies [27,28], although the molecular mechanisms remain largely unknown. Two recent in vitro studies on cardiomyocytes and endothelial cells suggest that the antiapoptotic effects of GHS are mediated by activation of both Akt and ERK kinases [29] and by regulating the activity of caspase-3 and expression of Bax and Bcl-2 [30]. These findings are largely in agreement with the mechanisms proposed in the present study.
ERK pathways are known to exert multiple functions in mammalian cell proliferation, cell death, cell survival, and neuronal plasticity [31,32], and ERK or CREB activation is necessary for memory function [32]. Our findings are consistent with a previous report that found treatment with a selective ERK inhibitor resulted in significant deficits in the novel object recognition test in mice [33]. CREB and ERK1/2 phosphorylation can be suppressed by sublethal Aβ treatment in cortical neuronal cells [34], and ERK 1/2 and CREB phosphorylation increases after paired conditioning in the mouse hippocampus [35]. These results indicated that the GH-releaser diet significantly attenuated klotho knockout-induced cognitive impairment mainly via the ERK/CREB pathway.
Another finding in the present study was that GSK3β phosphorylation was increased following the GH releaser diet, which is the expected response after activation of the PI3K/Akt pathway [36]. Activation of the PI3K pathway and the downstream phosphorylation of Akt are known to mediate growth factor-induced neuronal survival in vitro [37]. pAkt promotes cell survival and can inhibit apoptosis by inactivating several proapoptotic targets, including Bad, GSK3β, and caspase 9. GSK3β has been shown to play a role in apoptosis and to be a key target of the PI3K/Akt survival signaling pathway [38]. Therefore, inhibition of GSK3β through phosphorylation could partly explain the neuroprotective effect of the GH-releaser diet. Activation of GSK3β has been linked to the triggering of caspase-dependent apoptosis [39], and the GH releaser-induced reduction of GSK3β activity offers additional support for the involvement of caspases in klotho mutant mice.
Interestingly, the GH-releaser diet did not affect hippocampal IGF-1 and IGF-1 receptor expression; consequently, all parameters were examined in the wild-type mice. Our previous data [17,18] showed that serum IGF-1 and hippocampal IGF-1/IGF-1 receptor expressions were maintained at a physiological level in the Aβ (42-1)-treated (negative control) mice in spite of increases in the serum and pituitary GH levels evoked by the GH-releaser diet, suggesting that the levels of GH and IGF-1 were under the normal homeostatic regulation. However, it has been reported that serum GH levels of klotho mutant mice are similar to those of wild-type mice [21] even though the serum IGF-1 levels of klotho mutant mice are significantly lower than those of wild-type mice, indicating that homeostatic feedback regulation of GH and IGF-1 is impaired in the klotho mutant mice. Similar to our results, Kashimada et al. [40] demonstrated that repeated GH treatment for 5 weeks normalizes the IGF-1 mRNA levels in the liver of klotho mutant mice. Thus, restoring the normal homeostatic regulation of GH and IGF-1 might be one possible mechanism for the cognitive enhancing effect of the GH-releaser diet.
In conclusion, we demonstrated, for the first time, that the GH-releaser diet markedly attenuated cognitive impairment in klotho mutant mice via IGF-1-receptor stimulation. We provided evidence that the protective effect of a GH-releaser diet is at least partly mediated through the PI3-kinase pathway, including activation of Akt and inhibition of GSK3β through phosphorylation. We also suggest that the GH-releaser effect is partly mediated by the ERK pathways, including downstream phosphorylation CREB. These GH-releaser diet-mediated actions were significantly reversed by an IGF-1 receptor antagonist, suggesting a role of the IGF-I receptor. However, further studies will be required to clarify the molecular mechanism by which the GH-releaser diet-mediated IGF-1-receptor stimulation prevents the cognitive impairment.
Figures and Tables
Fig. 1
Experimental schedule for evaluating the effect of a growth hormone (GH)-releaser diet on the cognitive dysfunction in klotho mutant mice. Mice received a GH-releaser diet or a vehicle diet during the entire experimental period. JB-1, an insulin-like growth factor-1 (IGF-1) receptor antagonist, was infused (1 µg/5 µL/5 min, once daily for 25 days) into the lateral ventricle. Twenty days after the GH releaser diet began, a novel object recognition test (NORT) and a conditioned fear learning test (CFL) were performed. Mice were sacrificed for Western blot, reverse transcription-polymerase chain reaction (RT-PCR), or neurochemical analyses at 15 minutes after the CFL.
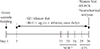
Fig. 2
Effect of a growth hormone (GH)-releaser diet on the mRNA expression of insulin-like growth factor-1 (IGF-1) and IGF-1 receptor in the hippocampus of klotho mutant mice. Each value is the mean±SEM of six animals. GAPDH, glyceraldehyde-3-phosphate dehydrogenase; aCSF, artificial cerebrospinal fluid; Veh, vehicle diet; GHR, GH-releaser diet. aP<0.01 vs. wild-type mice treated with aCSF and vehicle diet; bP<0.05; cP<0.01 vs. klotho mutant mice treated with aCSF and vehicle diet; dP<0.05; eP<0.01 vs. klotho mutant mice treated with aCSF and GH releaser diet (three-way analysis of variance followed by multiple pair-wise comparisons with Bonferroni's correction).
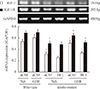
Fig. 3
Effect of JB-1, an insulin-like growth factor-1 (IGF-1) receptor antagonist, on the growth hormone (GH)-releaser diet-mediated attenuation of changes in the expression of (A) phospho-Akt (p-Akt), (B) phospho-glycogen synthase kinase3β (p-GSK3β), and (C) phospho-calcium/calmodulin-dependent kinase II (p-CaMKII) in the hippocampus of klotho mutant mice. Each value is the mean±SEM of six animals. aCSF, artificial cerebrospinal fluid; Veh, vehicle diet; GHR, GH-releaser diet. aP<0.01 vs. wild-type mice treated with aCSF and vehicle diet; bP<0.01 vs. klotho mutant mice treated with aCSF and vehicle diet; cP<0.05; dP<0.01 vs. klotho mutant mice treated with aCSF and a GH-releaser diet (three-way analysis of variance followed by multiple pair-wise comparisons with Bonferroni's correction).
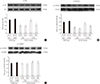
Fig. 4
Effect of JB-1, an insulin-like growth factor-1 (IGF-1) receptor antagonist, on the growth hormone (GH)-releaser diet-mediated attenuation of changes in the expression of (A) phospho-extracellular signal-related kinase (p-ERK), (B) phospho-c-jun N-terminal kinase (p-JNK), (C) phospho-p38 (p-p38), and (D) phospho-cAMP responsive element binding protein (p-CREB) in the hippocampus of klotho mutant mice. Each value is the mean±SEM of six animals. aCSF, artificial cerebrospinal fluid; Veh, vehicle diet; GHR, GH-releaser diet. aP<0.01 vs. wild-type mice treated with aCSF and vehicle diet; bP<0.05; cP<0.01 vs. klotho mutant mice treated with aCSF and vehicle diet; dP<0.05; eP<0.01 vs. klotho mutant mice treated with aCSF and a GH-releaser diet (three-way analysis of variance followed by multiple pair-wise comparisons with Bonferroni's correction).
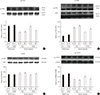
Fig. 5
Effect of JB-1, an insulin-like growth factor-1 (IGF-1) receptor antagonist, on the growth hormone (GH)-releaser diet-mediated attenuation of changes in the expression of (A) Bax, (B) cleaved caspase-3, (C) Bcl-2, and (D) Bcl-xL in the hippocampus of klotho mutant mice. Each value is the mean±SEM of six animals. aCSF, artificial cerebrospinal fluid; Veh, vehicle diet; GHR, GH-releaser diet. aP<0.01 vs. wild-type mice treated with aCSF and vehicle diet; bP<0.01 vs. klotho mutant mice treated with aCSF and vehicle diet; cP<0.05; dP<0.01 vs. klotho mutant mice treated with aCSF and a GH-releaser diet (three-way analysis of variance followed by multiple pair-wise comparisons with Bonferroni's correction).
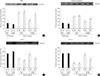
Fig. 6
Effect of JB-1, an insulin-like growth factor-1 (IGF-1) receptor antagonist, on the growth hormone (GH)-releaser diet-mediated attenuation of lipid peroxidation, as measured by (A) the malondialdehyde (MDA) level, and protein oxidation, as measured by (B) the protein carbonyl level in the hippocampus of klotho mutant mice. Each value is the mean±SEM of eight animals. DNPH, 2,4-dinitrophenylhydrazine; aCSF, artificial cerebrospinal fluid; Veh, vehicle diet; GHR, GH-releaser diet. aP<0.05; bP<0.01 vs. wild-type mice treated with aCSF and vehicle diet; cP<0.05; dP<0.01 vs. klotho mutant mice treated with aCSF and vehicle diet; eP<0.05 vs. klotho mutant mice treated with aCSF and a GH-releaser diet (three-way analysis of variance followed by multiple pair-wise comparisons with Bonferroni's correction).
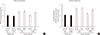
Fig. 7
Effect of JB-1, an insulin-like growth factor-1 (IGF-1) receptor antagonist, on the growth hormone (GH)-releaser diet-mediated attenuation of cognitive dysfunction, as evaluated by (A) a novel object recognition test and (B) a context-dependent conditioned fear learning test in klotho mutant mice. Each value is the mean±SEM of 10 animals. aCSF, artificial cerebrospinal fluid; Veh, vehicle diet; GHR, GH-releaser diet. aP<0.01 vs. wild-type mice treated with aCSF and vehicle diet; bP<0.01 vs. klotho mutant mice treated with aCSF and vehicle diet; cP<0.05 vs. klotho mutant mice treated with aCSF and a GH-releaser diet (three-way analysis of variance followed by multiple pair-wise comparisons with Bonferroni's correction).
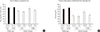
ACKNOWLEDGMENTS
This study was supported by a grant from the Brain Research Center from the 21st Century Frontier Research Program (2012K001115) funded by the Ministry of Science and Technology, Republic of Korea. JHL and DKD were supported by the BK21 PLUS program, National Research Foundation of Korea. Equipment at the Institute of Pharmaceutical Science (Kangwon National University) was used for this study.
References
1. Matsumura Y, Aizawa H, Shiraki-Iida T, Nagai R, Kuro-o M, Nabeshima Y. Identification of the human klotho gene and its two transcripts encoding membrane and secreted klotho protein. Biochem Biophys Res Commun. 1998; 242:626–630.
2. Shiraki-Iida T, Aizawa H, Matsumura Y, Sekine S, Iida A, Anazawa H, Nagai R, Kuro-o M, Nabeshima Y. Structure of the mouse klotho gene and its two transcripts encoding membrane and secreted protein. FEBS Lett. 1998; 424:6–10.
3. Kuro-o M. Klotho and aging. Biochim Biophys Acta. 2009; 1790:1049–1058.
4. Kuro-o M, Matsumura Y, Aizawa H, Kawaguchi H, Suga T, Utsugi T, Ohyama Y, Kurabayashi M, Kaname T, Kume E, Iwasaki H, Iida A, Shiraki-Iida T, Nishikawa S, Nagai R, Nabeshima YI. Mutation of the mouse klotho gene leads to a syndrome resembling ageing. Nature. 1997; 390:45–51.
5. Kuro-o M. Klotho as a regulator of fibroblast growth factor signaling and phosphate/calcium metabolism. Curr Opin Nephrol Hypertens. 2006; 15:437–441.
6. Kuro-o M. Klotho. Pflugers Arch. 2010; 459:333–343.
7. Kurosu H, Yamamoto M, Clark JD, Pastor JV, Nandi A, Gurnani P, McGuinness OP, Chikuda H, Yamaguchi M, Kawaguchi H, Shimomura I, Takayama Y, Herz J, Kahn CR, Rosenblatt KP, Kuro-o M. Suppression of aging in mice by the hormone Klotho. Science. 2005; 309:1829–1833.
8. Kuro-o M. Klotho as a regulator of oxidative stress and senescence. Biol Chem. 2008; 389:233–241.
9. Nagai T, Yamada K, Kim HC, Kim YS, Noda Y, Imura A, Nabeshima Y, Nabeshima T. Cognition impairment in the genetic model of aging klotho gene mutant mice: a role of oxidative stress. FASEB J. 2003; 17:50–52.
10. Anamizu Y, Kawaguchi H, Seichi A, Yamaguchi S, Kawakami E, Kanda N, Matsubara S, Kuro-o M, Nabeshima Y, Nakamura K, Oyanagi K. Klotho insufficiency causes decrease of ribosomal RNA gene transcription activity, cytoplasmic RNA and rough ER in the spinal anterior horn cells. Acta Neuropathol. 2005; 109:457–466.
11. Park SJ, Shin EJ, Min SS, An J, Li Z, Hee Chung Y, Hoon Jeong J, Bach JH, Nah SY, Kim WK, Jang CG, Kim YS, Nabeshima Y, Nabeshima T, Kim HC. Inactivation of JAK2/STAT3 signaling axis and downregulation of M1 mAChR cause cognitive impairment in klotho mutant mice, a genetic model of aging. Neuropsychopharmacology. 2013; 38:1426–1437.
12. Li SA, Watanabe M, Yamada H, Nagai A, Kinuta M, Takei K. Immunohistochemical localization of Klotho protein in brain, kidney, and reproductive organs of mice. Cell Struct Funct. 2004; 29:91–99.
13. Lamberts SW, van den Beld AW, van der Lely AJ. The endocrinology of aging. Science. 1997; 278:419–424.
14. van Dam PS, Aleman A, de Vries WR, Deijen JB, van der Veen EA, de Haan EH, Koppeschaar HP. Growth hormone, insulin-like growth factor I and cognitive function in adults. Growth Horm IGF Res. 2000; 10:Suppl B. S69–S73.
15. Khorram O. Use of growth hormone and growth hormone secretagogues in aging: help or harm. Clin Obstet Gynecol. 2001; 44:893–901.
16. Chromiak JA, Antonio J. Use of amino acids as growth hormone-releasing agents by athletes. Nutrition. 2002; 18:657–661.
17. Shin EJ, Jhoo JH, Nabeshima T, Jhoo WK, Kwon MS, Lim YK, Chae JS, Jung ME, Park SJ, Jang KJ, Kim HC. Growth hormone releaser attenuates beta-amyloid (1 - 42)-induced memory impairment in mice. J Pharmacol Sci. 2005; 99:117–120.
18. Shin EJ, Chae JS, Park SJ, Kim SC, Koo KH, Yamada K, Nabeshima T, Kim HC. Growth hormone-releaser diet attenuates beta-amyloid(1-42)-induced cognitive impairment via stimulation of the insulin-like growth factor (IGF)-1 receptor in mice. J Pharmacol Sci. 2009; 109:139–143.
19. Oliver CN, Ahn BW, Moerman EJ, Goldstein S, Stadtman ER. Age-related changes in oxidized proteins. J Biol Chem. 1987; 262:5488–5491.
20. Tran HY, Shin EJ, Saito K, Nguyen XK, Chung YH, Jeong JH, Bach JH, Park DH, Yamada K, Nabeshima T, Yoneda Y, Kim HC. Protective potential of IL-6 against trimethyltin-induced neurotoxicity in vivo. Free Radic Biol Med. 2012; 52:1159–1174.
21. Utsugi T, Ohno T, Ohyama Y, Uchiyama T, Saito Y, Matsumura Y, Aizawa H, Itoh H, Kurabayashi M, Kawazu S, Tomono S, Oka Y, Suga T, Kuro-o M, Nabeshima Y, Nagai R. Decreased insulin production and increased insulin sensitivity in the klotho mutant mouse, a novel animal model for human aging. Metabolism. 2000; 49:1118–1123.
22. Wolf I, Levanon-Cohen S, Bose S, Ligumsky H, Sredni B, Kanety H, Kuro-o M, Karlan B, Kaufman B, Koeffler HP, Rubinek T. Klotho: a tumor suppressor and a modulator of the IGF-1 and FGF pathways in human breast cancer. Oncogene. 2008; 27:7094–7105.
23. Yamamoto M, Clark JD, Pastor JV, Gurnani P, Nandi A, Kurosu H, Miyoshi M, Ogawa Y, Castrillon DH, Rosenblatt KP, Kuro-o M. Regulation of oxidative stress by the anti-aging hormone klotho. J Biol Chem. 2005; 280:38029–38034.
24. Chen CD, Podvin S, Gillespie E, Leeman SE, Abraham CR. Insulin stimulates the cleavage and release of the extracellular domain of Klotho by ADAM10 and ADAM17. Proc Natl Acad Sci U S A. 2007; 104:19796–19801.
25. Lorenzi O, Veyrat-Durebex C, Wollheim CB, Villemin P, Rohner-Jeanrenaud F, Zanchi A, Vischer UM. Evidence against a direct role of klotho in insulin resistance. Pflugers Arch. 2010; 459:465–473.
26. Frago LM, Paneda C, Dickson SL, Hewson AK, Argente J, Chowen JA. Growth hormone (GH) and GH-releasing peptide-6 increase brain insulin-like growth factor-I expression and activate intracellular signaling pathways involved in neuroprotection. Endocrinology. 2002; 143:4113–4122.
27. Locatelli V, Rossoni G, Schweiger F, Torsello A, De Gennaro Colonna V, Bernareggi M, Deghenghi R, Muller EE, Berti F. Growth hormone-independent cardioprotective effects of hexarelin in the rat. Endocrinology. 1999; 140:4024–4031.
28. Tivesten A, Bollano E, Caidahl K, Kujacic V, Sun XY, Hedner T, Hjalmarson A, Bengtsson BA, Isgaard J. The growth hormone secretagogue hexarelin improves cardiac function in rats after experimental myocardial infarction. Endocrinology. 2000; 141:60–66.
29. Baldanzi G, Filigheddu N, Cutrupi S, Catapano F, Bonissoni S, Fubini A, Malan D, Baj G, Granata R, Broglio F, Papotti M, Surico N, Bussolino F, Isgaard J, Deghenghi R, Sinigaglia F, Prat M, Muccioli G, Ghigo E, Graziani A. Ghrelin and des-acyl ghrelin inhibit cell death in cardiomyocytes and endothelial cells through ERK1/2 and PI 3-kinase/AKT. J Cell Biol. 2002; 159:1029–1037.
30. Pang JJ, Xu RK, Xu XB, Cao JM, Ni C, Zhu WL, Asotra K, Chen MC, Chen C. Hexarelin protects rat cardiomyocytes from angiotensin II-induced apoptosis in vitro. Am J Physiol Heart Circ Physiol. 2004; 286:H1063–H1069.
31. English JD, Sweatt JD. Activation of p42 mitogen-activated protein kinase in hippocampal long term potentiation. J Biol Chem. 1996; 271:24329–24332.
32. Atkins CM, Selcher JC, Petraitis JJ, Trzaskos JM, Sweatt JD. The MAPK cascade is required for mammalian associative learning. Nat Neurosci. 1998; 1:602–609.
33. Nagai T, Takuma K, Kamei H, Ito Y, Nakamichi N, Ibi D, Nakanishi Y, Murai M, Mizoguchi H, Nabeshima T, Yamada K. Dopamine D1 receptors regulate protein synthesis-dependent long-term recognition memory via extracellular signal-regulated kinase 1/2 in the prefrontal cortex. Learn Mem. 2007; 14:117–125.
34. Tong L, Balazs R, Thornton PL, Cotman CW. Beta-amyloid peptide at sublethal concentrations downregulates brain-derived neurotrophic factor functions in cultured cortical neurons. J Neurosci. 2004; 24:6799–6809.
35. Trifilieff P, Herry C, Vanhoutte P, Caboche J, Desmedt A, Riedel G, Mons N, Micheau J. Foreground contextual fear memory consolidation requires two independent phases of hippocampal ERK/CREB activation. Learn Mem. 2006; 13:349–358.
36. Cross DA, Alessi DR, Cohen P, Andjelkovich M, Hemmings BA. Inhibition of glycogen synthase kinase-3 by insulin mediated by protein kinase B. Nature. 1995; 378:785–789.
37. Kulik G, Klippel A, Weber MJ. Antiapoptotic signalling by the insulin-like growth factor I receptor, phosphatidylinositol 3-kinase, and Akt. Mol Cell Biol. 1997; 17:1595–1606.
38. Pap M, Cooper GM. Role of glycogen synthase kinase-3 in the phosphatidylinositol 3-Kinase/Akt cell survival pathway. J Biol Chem. 1998; 273:19929–19932.
39. Watcharasit P, Bijur GN, Zmijewski JW, Song L, Zmijewska A, Chen X, Johnson GV, Jope RS. Direct, activating interaction between glycogen synthase kinase-3beta and p53 after DNA damage. Proc Natl Acad Sci U S A. 2002; 99:7951–7955.
40. Kashimada K, Yamashita T, Tsuji K, Nifuji A, Mizutani S, Nabeshima Y, Noda M. Defects in growth and bone metabolism in klotho mutant mice are resistant to GH treatment. J Endocrinol. 2002; 174:403–410.