Abstract
The sweet taste receptors present in the taste buds are heterodimers comprised of T1R2 and T1R3. This receptor is also expressed in pancreatic β-cells. When the expression of receptor subunits is determined in β-cells by quantitative reverse transcription polymerase chain reaction, the mRNA expression level of T1R2 is extremely low compared to that of T1R3. In fact, the expression of T1R2 is undetectable at the protein level. Furthermore, knockdown of T1R2 does not affect the effect of sweet molecules, whereas knockdown of T1R3 markedly attenuates the effect of sweet molecules. Consequently, a homodimer of T1R3 functions as a receptor sensing sweet molecules in β-cells, which we designate as sweet taste-sensing receptors (STSRs). Various sweet molecules activate STSR in β-cells and augment insulin secretion. With regard to intracellular signals, sweet molecules act on STSRs and increase cytoplasmic Ca2+ and/or cyclic AMP (cAMP). Specifically, when an STSR is stimulated by one of four different sweet molecules (sucralose, acesulfame potassium, sodium saccharin, or glycyrrhizin), distinct signaling pathways are activated. Patterns of changes in cytoplasmic Ca2+ and/or cAMP induced by these sweet molecules are all different from each other. Hence, sweet molecules activate STSRs by acting as biased agonists.
Sweet taste receptor expressed in the taste buds is a heterodimer comprised of two members of the class C G protein-coupled receptors, T1R2 and T1R3. These subunits are also expressed in extragustatory organs including small intestine, pancreatic islets and adipocytes. In pancreatic islets, T1R3 is dominantly expressed and a homodimer of T1R3 may function as a sweet taste-sensing receptor. In this article, the signal transduction systems activated by sweet molecules in pancreatic β-cells are presented.
Nutrient sensing is quite important for maintaining energy homeostasis in living organisms. In mammals, the taste of nutrients is detected by a special apparatus in the oral cavity, i.e., the taste bud, where various types of taste cells are located. With regard to sweet taste, detection of sweet molecules is achieved by the sweet taste receptor present on the tips of taste cells. The molecular nature of the sweet taste receptor was identified more than a decade ago by several groups [1,2,3,4,5,6]. Nelson et al. [3], Zhang et al. [7], and Zhao et al. [8] have demonstrated that T1R2 and T1R3, both of which are class C members of the G protein-coupled receptor (GPCR) family, form a heterodimer and function as the sweet taste receptor. Identification of the sweet taste receptor then raised the simple question as to whether or not both low molecular weight sweeteners and large molecular weight sweet proteins bind to the same receptors and activate taste cells. Subsequently, it was shown that various sweet molecules with small to large molecular weights do interact with the same receptors [9]. Thus, the human sweet taste receptor is activated by sugars such as fructose, galactose, glucose, maltose, and sucrose and also by amino acids including glycine, alanine, threonine, D-tryptophan, and D-histidine. These receptors are also activated by the dipeptide L-aspartyl-L-pheylatanine and sweet proteins such as monellin, thaumatin, and brazzein [10]. Furthermore, these receptors are activated by a large number of natural and artificial sweeteners with diverse chemical structures [10]. Collectively, the sweet taste receptor has a tremendous capacity to bind various sweet molecules, resulting in transmission of the ligand signal to the cell interior and eventual activation of the taste cells. Activated taste cells then release adenosine triphosphate (ATP), which stimulates purinergic P2X receptors expressed in afferent neural fibers. Studies using knockout mice have revealed that most of the sweet sensation is mediated by the heterodimer of T1R2 and T1R3 [3,7,8]. However, a small amount of nerve activity was observed in response to sugars in T1R3 knockout mice [11]. This raises the possibility of an additional mechanism for detection of sweet molecules. It seems likely that an alternate sweet-sensing activity is mediated by different oligomerizations of related GPCRs.
The signal transduction pathway activated by the sweet taste receptor has been studied extensively. In particular, studies using knockout mice have revealed the major signaling pathway activated by the sweet taste receptor. When activated by sweet molecules, the signal is transmitted to a taste cell-specific trimeric G protein, gustducin (Ggust) [12], and subsequent activation of phospholipase C-β2 (PLC-β2) leads to the generation of two messengers, inositol 1,4,5-trisphosphate (Ins-P3) and diacylglycerol [13]. The former activates the Ins-P3 receptor channel in the endoplasmic reticulum (ER) and induces Ca2+ release from ER, which in turn activates the TRPM5 cation channel in the plasma membrane [14]. The resultant influx of Na+ causes depolarization of the plasma membrane, which induces a release of ATP through voltage-dependent calcium homeostasis modulator-1 [15]. TRPM5 is a sodium-permeable cation channel, and its activity depends on the temperature [14]. This explains the fact that sweet taste-sensing is diminished at low temperatures. Note that this signaling pathway was established largely based on data obtained in in vivo animal experiments by monitoring activation of sensory nerves as an output. Since the measurement of intracellular signals in isolated taste cells or taste cells in situ is rather difficult, the precise signaling mechanism is not elucidated at the cellular level. It is not certain whether a signaling pathway other than the gustducin-PLC-β2 pathway is involved in sweet taste-sensing. In this regard, α-gustducin knockout mice are able to sense sweet flavors [16,17], suggesting that an alternate pathway is also involved in the recognition of the sweet sensation. It has been reported that cyclic AMP (cAMP) is increased in taste buds after stimulation by sweet molecules [18]. Nevertheless, it is not certain whether the sweet taste receptor is also coupled to the Gs-cAMP cascade.
The sweet taste receptor is activated by various sweet molecules with diverse chemical structures [10]. Many studies have been conducted to identify the binding sites for sweet molecules and the mode of activation by those ligands. The similarity of mGluR1 and the sweet taste receptor has helped to build models of ligand binding to the sweet taste receptor. The amino-terminal domain (ATD) of the homodimer of mGluR1 is composed of two lobes, LB1 and LB2, and the glutamate-binding domain is located between LB1 and LB2. The ATD of mGuR1 exists in an equilibrium of two different conformations, the structures of which depend on the binding of glutamate. In the ligand-free state, LB1 and LB2 show open conformations (open-open). Binding of glutamate induces the closed conformation of LB1 and LB2 in one ATD, whereas the others remain in an open conformation. The closed-open structure is characteristic of the active state of mGluR1 [18]. It should be noted that the sweet taste receptor is activated by small molecules and also by large proteins. In this regard, Temussi [19] proposed a wedge model using the information obtained with mGluR1 [20], in which protein sweeteners bind to the secondary binding site on the surface of the receptor and act as a wedge. It is now thought that there are multiple binding sites of different dimensions in the ATD domain for small and large nonprotein sweeteners [21]. The ATD of T1R2 is responsible for the binding of aspartame and sugar analogues such as sucralose [22], as well as that of acesulfame potassium (acesulfame-K) and sodium saccharin (saccharin-Na) [23]. In addition, cyclamate and lactisole, an inhibitor of human T1R3, bind to the transmembrane domain of T1R3 [24], which may also function as an allosteric binding site for saccharin [25]. There is a possibility that the cysteine-rich domain offers another binding domain for protein sweeteners [26,27].
The sweet taste receptor is activated by various types of sweet molecules with diverse chemical structures, which bind to multiple sites in the receptor. It is, however, not certain whether all these agonists induce a common cellular response. When T1R2 and T1R3 were expressed heterologously in HEK293 cells, the sweeteners examined increased the cytoplasmic free calcium concentration ([Ca2+]c) [7]. It was not determined whether these agonists induced the production of other second messengers, for example, cAMP. Those studies were conducted in cells heterologously expressing T1R2 and T1R3, but the number of heterologously expressed receptors may have been greatly different from that in cells expressing the endogenous receptor. Furthermore, an artificial chimeric G protein was introduced as a transducer to observe the activation of PLC. It is possible that the arrangement of signaling molecules in or near the plasma membrane was quite different compared to that in taste cells. It is not certain whether various sweet molecules induce a uniform cellular response in cells expressing the endogenous sweet taste receptor.
It is now known that the sweet taste receptor is expressed in cells of extragustatory organs, including neuroendocrine cells in the gastrointestinal tract [28,29,30], glucose-responsive neurons in the hypothalamus [31], adipocytes [32], epithelial cells of the urinary bladder [33], and chemosensory cells in the airway [34].
We have shown that the sweet taste receptor is expressed in pancreatic β-cells and in an insulin-secreting cell line, MIN6 [35]. When this receptor is activated by the artificial sweetener sucralose in the presence of 2.7 mM glucose, insulin secretion is augmented, indicating that the sweet taste receptor is functional [35]. With regard to the expression of the receptor subunits, mRNA for T1R2 and T1R3 have been detected by reverse transcription polymerase chain reaction (RT-PCR) both in mouse islets and in MIN6 cells [35]. However, when the expression of T1Rs was measured by quantitative RT-PCR, the expression level of T1R2 was quite low compared to that of T1R3 [36]. In addition, the protein expression of T1R2 was not detected by either immunoblotting or immunohistochemistry. Furthermore, knockdown of T1R3 attenuates the effect of sucralose, whereas knockdown of T1R2 does not affect the response to sucralose [37]. These observations indicate that the sweet taste receptor in β-cells may be a homodimer of T1R3 rather than a heterodimer of T1R2 and T1R3, as has been previously thought (Fig. 1). Consequently, the sweet taste receptor expressed in pancreatic β-cells is slightly different from the cannonical sweet taste receptor system in taste cells. We designated the receptor expressed in β-cells as the sweet taste-sensing receptor (STSR). It should be noted that we have not excluded the possibility that T1R3 forms a heterodimer with other members of class C GPCRs. Although it has been generally thought that T1Rs form a heterodimer and function as sweet or umami taste receptors [7,8,10], recent studies have revealed that a homodimer of T1R3 also functions as a taste receptor. Thus, a homodimer of human T1R3 acts as a calcium taste-sensing receptor [38]. In addition, a homodimer of mouse T1R3 is activated by sucralose and saccharin-Na in 3T3-L1 adipocytes [32]. Moreover, sucralose activates the mouse T1R3 homodimer in HEK293 cells heterologously expressing T1R3 [32]. It is thus possible that, in some types of cells, especially those in extragustatory organs, a homodimer of T1R3 functions as a STSR.
When STSRs in MIN6 cells are activated by sucralose, an artificial sugar analogue, there is an immediate increase in [Ca2+]c, which is followed by sustained elevation of [Ca2+]c [35]. Sucralose also elicits a rapid and sustained elevation of cytoplasmic cAMP concentration ([cAMP]c) [35]. Accordingly, STSRs expressed in pancreatic β-cells activate dual signaling pathways, namely the Ca2+ and cAMP signaling systems. It should be noted that the doses of sucralose needed to induce Ca2+ and cAMP signals are relatively high. This may be due to the fact that STSR is a homodimer of T1R3.
MIN6 cells thus provide a suitable cell system to precisely investigate the intracellular signaling pathways activated by endogenous STSR. Using this system, we have addressed the question of whether various sweet molecules induce a uniform cellular response. We have examined the effects of four sweeteners, sucralose, acesulfame-K, saccharin-Na, and dipotassium glycyrrhizinate (DPG), and compared the actions of these sweeteners on [Ca2+]c and [cAMP]. With regard to the ligand binding site, a recent study has shown that sucralose, acesulfame-K, and saccharin-Na bind to the ATD of T1R2 [23]. Saccharin-Na may also interact with the transmembrane domain of T1R3. The binding site for DPG has not yet been identified. However, since STSRs in β-cells are homodimers of T1R3 and do not involve T1R2, exact binding sites for sucralose, acesulfame-K, and saccharin-Na are not certain in β-cells. Perhaps they bind to some portions of the ATD of T1R3. In any case, the effects of these four sweeteners are herein examined in MIN6 cells [37]. When we measure insulin secretion, all four sweeteners significantly increase insulin secretion, and acesulfame-K is the most potent stimulator of secretion [37]. In terms of the final output of the cells, i.e., insulin secretion, the four sweeteners elicit qualitatively similar effects. However, when we measure intracellular events evoked by the four sweeteners, the actions of these four sweeteners are quite different. Fig. 2 shows typical responses of [Ca2+]c and [cAMP]c to the four sweeteners. Saccharin-Na and DPG increase the expression of one of the two messengers [37]. Specifically, saccharin-Na increases [cAMP]c but does not affect [Ca2+]c, whereas DPG increases [Ca2+]c without inducing significant changes in [cAMP]c. In contrast, sucralose and acesulfame-K elevated both [Ca2+]c and [cAMP]c. Although these two sweeteners increase both [Ca2+]c and [cAMP]c, their actions are different. For example, gurmarin, which attenuates sweet taste sensation [39], inhibits the effect of sucralose on [Ca2+]c, whereas the effect of acesulfame-K is resistant to gurmarin. Furthermore, a Gq inhibitor YM254890 [40] markedly inhibits [Ca2+]c response to sucralose, whereas YM254890 has little effect on acesulfame-K-induced elevation of [Ca2+]c. Collectively, the effects of the four sweeteners on [Ca2+]c and [cAMP]c differ in many respects [37]. Although the intracellular responses elicited by the four sweeteners are quite different, all of their actions are mediated by STSRs since knockdown of T1R3 markedly inhibits intracellular responses to the four sweeteners [37]. These results clearly indicate that the four sweeteners act as biased agonists and activate distinct signaling pathways.
Among the four agonists, sucralose, acesulfame-K, and saccharin-Na increase [cAMP]c. Interestingly, the effects of these three agonists on [cAMP]c are once again not the same. Knockdown of Gs significantly attenuates the elevation of [cAMP]c induced by sucralose and saccharin-Na, indicating that Gs is involved in the actions of these two sweeteners [37]. This is the first demonstration of the involvement of Gs in the signaling system mediated by T1R3. In contrast, the effect of acesulfame-K is not significantly attenuated by knockdown of Gs. Instead, the effect of acesulfame-K on [cAMP]c is blocked by removal of extracellular calcium. It is well known that calcium-calmodulin-dependent adenylyl cyclase (AC) is expressed in pancreatic β-cells [41]. Presumably, marked elevation of [Ca2+]c induced by acesulfame-K would activate calcium-dependent AC, in particular AC VIII, and generate cAMP. This subtype of AC is also activated by Gs [42]. Therefore, both Ca2+ and Gs activate AC VIII when cells are stimulated by sucralose. In any case, the mechanism for the production of cAMP is different depending upon the type of agonist.
With regard to the Ca2+ signal, sucralose, acesulfame-K, and DPG increase [Ca2+]c by causing both Ca2+ release from an intracellular pool and stimulation of Ca2+ entry. Ca2+ release is mediated by activation of PLC, as demonstrated by the attenuation of [Ca2+]c responses to the three agonists by an inhibitor of PLC [37]. STSR may activate PLC by a G-protein-dependent mechanism. The G protein involved in the activation of PLC may be different depending on the type of agonist. YM254890, a compound that inhibits Gq, G11, and G14 [40], attenuates the action of sucralose, but those of acesulfame-K and DPG are resistant to YM254890. G proteins involved in the activation of PLC are different with regard to the actions of the three sweeteners. Similarly, Ca2+ entry induced by the three sweeteners is not the same. Ca2+ entry induced by sucralose and acesulfame-K is dependent on extracellular Na+, whereas that induced by DPG is relatively resistant to removal of extracellular Na+. Presumably, as we mentioned previously [35], sucralose and acesulfame-K stimulate sodium entry, cause depolarization and thereby activate the voltage-dependent Ca2+ channel. In contrast, DPG may activate a Ca2+-permeable channel and induce Ca2+ entry. Ca2+ enters the β-cells through different types of Ca2+-permeable channels. Fig. 3 depicts a schematic presentation of the action of the four agonists on [Ca2+]c and [cAMP]c. As can be seen, although the four sweet agonists bind to STSR (T1R3 homodimer), the four agonists activate different sets of G proteins [43] and subsequent effector molecules. Hence, these sweet agonists act as biased agonists activating different sets of signaling cascades. This notion is in sharp contrast to the conventional view that sweet molecules bind to the sweet taste receptor to uniformly activate the Ggust-PLC-β2-TRPM5 pathway [44].
The fact that sweet molecules act as biased agonists raises several additional questions. First, do many other agonists for STSR in β-cells act similarly and activate one of the signaling pathways described in Fig. 3, or do they activate an additional distinct signaling cascade? Of particular interest is the effect of sweet proteins since they bind to a different portion of the receptor, the cysteine-rich domain, compared to the four agonists already examined. This question should be addressed experimentally. Second, do various agonists for STSR produce identical cellular output? As mentioned above, all of the four sweeteners stimulate insulin secretion. When different outputs are measured, however, the four sweeteners may exert different cellular outputs in MIN6 cells. In fact, when we assessed the apoptosis-inhibiting action of the sweet agonists, both sucralose and saccharin-Na prevented apoptosis induced by ER stress in MIN6 cells, whereas acesulfame-K and DPG had little effect on apoptosis. If we measure various cellular outputs, sweet molecules elicit qualitatively different effects. Third, do various sweet molecules act as biased agonists in cells other than β-cells? This is an interesting issue for which we already have some answers. In adipocytes, sucralose, by acting on STSRs (homodimers of T1R3), increases [cAMP]c but does not affect [Ca2+]c [32]. The effect of sucralose in adipocytes is apparently different from that in β-cells. Given that biased agonism occurs in a cell-type specific manner [43], sweet molecules also act as biased agonists in adipocytes. Since STSR is expressed in nongustatory systems, additional studies are required to elucidate the variety of functions of this receptor in other types of cells. Then, an additional question arises as to whether sweet molecules act as biased agonists in taste cells of the taste bud. This question is difficult to answer since the sweet taste receptor expressed in the taste bud is composed of T1R2 and T1R3, which is slightly different from STSRs present in nongustatory cells such as β-cells and adipocytes. It is, however, possible that the sweet taste receptors in taste cells function as STSRs in β-cells. If so, our understanding of the mechanism for sweet taste sensation should be revised extensively. Experiments conducted in taste cells are definitely needed to address this issue.
In summary, STSRs expressed in β-cells showed great flexibility in generating multiple patterns of intracellular signals. A major function of β-cells is to detect nutritional signals in the plasma including sugars, amino acid and lipids and to secrete insulin. In this regard, STSRs detect changes in sugar and amino acid levels and produce a variety of intracellular signals. STSRs may play an important physiological role in β-cell functions. Studies are now in progress in our laboratory to elucidate the physiological significance of STSRs in β-cells.
Figures and Tables
Fig. 1
Comparison of (A) the sweet taste receptor in taste cells and (B) the sweet taste-sensing receptor in β-cells. The sweet taste receptor expressed in taste cells of the taste bud (left) is a heterodimer comprised of T1R2 and T1R3, whereas the sweet taste-sensing receptor expressed in β-cells (right) is a homodimer of T1R3.
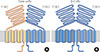
Fig. 2
Comparison of the effects of four sweeteners on [Ca2+]c and [cyclic AMP, cAMP]c. MIN6 cells were stimulated by (A) 50-mM acesulfame-K, (B) 50-mM sucralose, (C) 3-mM dipotassium glycyrrhizinate (DPG), or (D) 50-mM saccharin-Na as indicated by the arrows. Changes in [Ca2+]c (○) and [cAMP]c (●) were monitored.
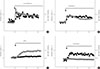
Fig. 3
Schematic presentation of the actions of the four sweeteners. Signaling cascades activated by (A) acesulfame-K, (B) sucralose, (C) dipotassium glycyrrhizinate (DPG), and (D) saccharin-Na are shown. When the colors of the G proteins and channels are different, it means that the molecules are different. AC, adenylyl cyclase; PLC, phospholipase C; αS, α-subunit of Gs; αX, α-subunit of YM254890-sensitive G protein; αY, α-subunit of YM254890-insensitive G protein; ATP, adenosine triphosphate; cAMP, cyclic AMP; PI(4,5)P2, phosphatidylinositol 4,5-bisphosphate; DAG, diacylglycerol; INsP3, inositol 1,4,5-tripsphophate.
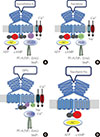
ACKNOWLEDGMENTS
The authors are grateful to Mayumi Odagiri for her secretarial assistance. This study was supported by Grant-in-Aid from the Ministry of Education, Sports and Culture of Japan.
References
1. Bachmanov AA, Li X, Reed DR, Ohmen JD, Li S, Chen Z, Tordoff MG, de Jong PJ, Wu C, West DB, Chatterjee A, Ross DA, Beauchamp GK. Positional cloning of the mouse saccharin preference (Sac) locus. Chem Senses. 2001; 26:925–933.
2. Li X, Inoue M, Reed DR, Huque T, Puchalski RB, Tordoff MG, Ninomiya Y, Beauchamp GK, Bachmanov AA. High-resolution genetic mapping of the saccharin preference locus (Sac) and the putative sweet taste receptor (T1R1) gene (Gpr70) to mouse distal Chromosome 4. Mamm Genome. 2001; 12:13–16.
3. Nelson G, Hoon MA, Chandrashekar J, Zhang Y, Ryba NJ, Zuker CS. Mammalian sweet taste receptors. Cell. 2001; 106:381–390.
4. Kitagawa M, Kusakabe Y, Miura H, Ninomiya Y, Hino A. Molecular genetic identification of a candidate receptor gene for sweet taste. Biochem Biophys Res Commun. 2001; 283:236–242.
5. Max M, Shanker YG, Huang L, Rong M, Liu Z, Campagne F, Weinstein H, Damak S, Margolskee RF. Tas1r3, encoding a new candidate taste receptor, is allelic to the sweet responsiveness locus Sac. Nat Genet. 2001; 28:58–63.
6. Montmayeur JP, Liberles SD, Matsunami H, Buck LB. A candidate taste receptor gene near a sweet taste locus. Nat Neurosci. 2001; 4:492–498.
7. Zhang Y, Hoon MA, Chandrashekar J, Mueller KL, Cook B, Wu D, Zuker CS, Ryba NJ. Coding of sweet, bitter, and umami tastes: different receptor cells sharing similar signaling pathways. Cell. 2003; 112:293–301.
8. Zhao GQ, Zhang Y, Hoon MA, Chandrashekar J, Erlenbach I, Ryba NJ, Zuker CS. The receptors for mammalian sweet and umami taste. Cell. 2003; 115:255–266.
9. Li X, Staszewski L, Xu H, Durick K, Zoller M, Adler E. Human receptors for sweet and umami taste. Proc Natl Acad Sci U S A. 2002; 99:4692–4696.
10. Temussi P. The sweet taste receptor: a single receptor with multiple sites and modes of interaction. Adv Food Nutr Res. 2007; 53:199–239.
11. Damak S, Rong M, Yasumatsu K, Kokrashvili Z, Varadarajan V, Zou S, Jiang P, Ninomiya Y, Margolskee RF. Detection of sweet and umami taste in the absence of taste receptor T1r3. Science. 2003; 301:850–853.
12. McLaughlin SK, McKinnon PJ, Margolskee RF. Gustducin is a taste-cell-specific G protein closely related to the transducins. Nature. 1992; 357:563–569.
13. Rössler P, Kroner C, Freitag J, Noe J, Breer H. Identification of a phospholipase C beta subtype in rat taste cells. Eur J Cell Biol. 1998; 77:253–261.
14. Prawitt D, Monteilh-Zoller MK, Brixel L, Spangenberg C, Zabel B, Fleig A, Penner R. TRPM5 is a transient Ca2+-activated cation channel responding to rapid changes in [Ca2+]i. Proc Natl Acad Sci U S A. 2003; 100:15166–15171.
15. Taruno A, Vingtdeux V, Ohmoto M, Ma Z, Dvoryanchikov G, Li A, Adrien L, Zhao H, Leung S, Abernethy M, Koppel J, Davies P, Civan MM, Chaudhari N, Matsumoto I, Hellekant G, Tordoff MG, Marambaud P, Foskett JK. CALHM1 ion channel mediates purinergic neurotransmission of sweet, bitter and umami tastes. Nature. 2013; 495:223–226.
16. Ruiz CJ, Wray K, Delay E, Margolskee RF, Kinnamon SC. Behavioral evidence for a role of alpha-gustducin in glutamate taste. Chem Senses. 2003; 28:573–579.
17. He W, Yasumatsu K, Varadarajan V, Yamada A, Lem J, Ninomiya Y, Margolskee RF, Damak S. Umami taste responses are mediated by alpha-transducin and alpha-gustducin. J Neurosci. 2004; 24:7674–7680.
18. Trubey KR, Culpepper S, Maruyama Y, Kinnamon SC, Chaudhari N. Tastants evoke cAMP signal in taste buds that is independent of calcium signaling. Am J Physiol Cell Physiol. 2006; 291:C237–C244.
19. Temussi PA. Why are sweet proteins sweet? Interaction of brazzein, monellin and thaumatin with the T1R2-T1R3 receptor. FEBS Lett. 2002; 526:1–4.
20. Kunishima N, Shimada Y, Tsuji Y, Sato T, Yamamoto M, Kumasaka T, Nakanishi S, Jingami H, Morikawa K. Structural basis of glutamate recognition by a dimeric metabotropic glutamate receptor. Nature. 2000; 407:971–977.
21. Xu H, Staszewski L, Tang H, Adler E, Zoller M, Li X. Different functional roles of T1R subunits in the heteromeric taste receptors. Proc Natl Acad Sci U S A. 2004; 101:14258–14263.
22. Cui M, Jiang P, Maillet E, Max M, Margolskee RF, Osman R. The heterodimeric sweet taste receptor has multiple potential ligand binding sites. Curr Pharm Des. 2006; 12:4591–4600.
23. Masuda K, Koizumi A, Nakajima K, Tanaka T, Abe K, Misaka T, Ishiguro M. Characterization of the modes of binding between human sweet taste receptor and low-molecular-weight sweet compounds. PLoS One. 2012; 7:e35380.
24. Jiang P, Cui M, Zhao B, Snyder LA, Benard LM, Osman R, Max M, Margolskee RF. Identification of the cyclamate interaction site within the transmembrane domain of the human sweet taste receptor subunit T1R3. J Biol Chem. 2005; 280:34296–34305.
25. Galindo-Cuspinera V, Winnig M, Bufe B, Meyerhof W, Breslin PA. A TAS1R receptor-based explanation of sweet 'water-taste'. Nature. 2006; 441:354–357.
26. Jiang P, Ji Q, Liu Z, Snyder LA, Benard LM, Margolskee RF, Max M. The cysteine-rich region of T1R3 determines responses to intensely sweet proteins. J Biol Chem. 2004; 279:45068–45075.
27. Masuda T, Taguchi W, Sano A, Ohta K, Kitabatake N, Tani F. Five amino acid residues in cysteine-rich domain of human T1R3 were involved in the response for sweet-tasting protein, thaumatin. Biochimie. 2013; 95:1502–1505.
28. Dyer J, Salmon KS, Zibrik L, Shirazi-Beechey SP. Expression of sweet taste receptors of the T1R family in the intestinal tract and enteroendocrine cells. Biochem Soc Trans. 2005; 33(Pt 1):302–305.
29. Bezençon C, le Coutre J, Damak S. Taste-signaling proteins are coexpressed in solitary intestinal epithelial cells. Chem Senses. 2007; 32:41–49.
30. Kojima I, Nakagawa Y. The role of the sweet taste receptor in enteroendocrine cells and pancreatic beta-cells. Diabetes Metab J. 2011; 35:451–457.
31. Ren X, Zhou L, Terwilliger R, Newton SS, de Araujo IE. Sweet taste signaling functions as a hypothalamic glucose sensor. Front Integr Neurosci. 2009; 3:12.
32. Masubuchi Y, Nakagawa Y, Ma J, Sasaki T, Kitamura T, Yamamoto Y, Kurose H, Kojima I, Shibata H. A novel regulatory function of sweet taste-sensing receptor in adipogenic differentiation of 3T3-L1 cells. PLoS One. 2013; 8:e54500.
33. Elliott RA, Kapoor S, Tincello DG. Expression and distribution of the sweet taste receptor isoforms T1R2 and T1R3 in human and rat bladders. J Urol. 2011; 186:2455–2462.
34. Tizzano M, Cristofoletti M, Sbarbati A, Finger TE. Expression of taste receptors in solitary chemosensory cells of rodent airways. BMC Pulm Med. 2011; 11:3.
35. Nakagawa Y, Nagasawa M, Yamada S, Hara A, Mogami H, Nikolaev VO, Lohse MJ, Shigemura N, Ninomiya Y, Kojima I. Sweet taste receptor expressed in pancreatic beta-cells activates the calcium and cyclic AMP signaling systems and stimulates insulin secretion. PLoS One. 2009; 4:e5106.
36. Kyriazis GA, Soundarapandian MM, Tyrberg B. Sweet taste receptor signaling in beta cells mediates fructose-induced potentiation of glucose-stimulated insulin secretion. Proc Natl Acad Sci U S A. 2012; 109:E524–E532.
37. Nakagawa Y, Nagasawa M, Mogami H, Lohse M, Ninomiya Y, Kojima I. Multimodal function of the sweet taste receptor expressed in pancreatic beta-cells: generation of diverse patterns of intracellular signals by sweet agonists. Endocr J. 2013; 60:1191–1206.
38. Tordoff MG, Alarcon LK, Valmeki S, Jiang P. T1R3: a human calcium taste receptor. Sci Rep. 2012; 2:496.
39. Imoto T, Miyasaka A, Ishima R, Akasaka K. A novel peptide isolated from the leaves of Gymnema sylvestre. I. Characterization and its suppressive effect on the neural responses to sweet taste stimuli in the rat. Comp Biochem Physiol A Comp Physiol. 1991; 100:309–314.
40. Nakagawa Y, Ohtsu Y, Nagasawa M, Shibata H, Kojima I. Glucose promotes its own metabolism by acting on the cell-surface glucose-sensing receptor T1R3. Endocr J. 2013; 11. 07. Epub DOI: http://dx.doi.org/10.1507/endocrj.EJ13-0431.
41. Furman B, Ong WK, Pyne NJ. Cyclic AMP signaling in pancreatic islets. Adv Exp Med Biol. 2010; 654:281–304.
42. Cali JJ, Zwaagstra JC, Mons N, Cooper DM, Krupinski J. Type VIII adenylyl cyclase. A Ca2+/calmodulin-stimulated enzyme expressed in discrete regions of rat brain. J Biol Chem. 1994; 269:12190–12195.
43. Kenakin TP. Biased signalling and allosteric machines: new vistas and challenges for drug discovery. Br J Pharmacol. 2012; 165:1659–1669.
44. Roper SD. Signal transduction and information processing in mammalian taste buds. Pflugers Arch. 2007; 454:759–776.