Abstract
Background
Panax ginseng has glucose-lowering effects, some of which are associated with the improvement in insulin resistance in skeletal muscle. Because mitochondria play a pivotal role in the insulin resistance of skeletal muscle, we investigated the effects of the ginsenoside Rg3, one of the active components of P. ginseng, on mitochondrial function and biogenesis in C2C12 myotubes.
Methods
C2C12 myotubes were treated with Rg3 for 24 hours. Insulin signaling pathway proteins were examined by Western blot. Cellular adenosine triphosphate (ATP) levels and the oxygen consumption rate were measured. The protein or mRNA levels of mitochondrial complexes were evaluated by Western blot and quantitative reverse transcription polymerase chain reaction analysis.
Results
Rg3 treatment to C2C12 cells activated the insulin signaling pathway proteins, insulin receptor substrate-1 and Akt. Rg3 increased ATP production and the oxygen consumption rate, suggesting improved mitochondrial function. Rg3 increased the expression of peroxisome proliferator-activated receptor γ coactivator 1α, nuclear respiratory factor 1, and mitochondrial transcription factor, which are transcription factors related to mitochondrial biogenesis. Subsequent increased expression of mitochondrial complex IV and V was also observed.
There are diverse types of ginseng, and it has been reported that some types improve blood glucose levels in patients with type 2 diabetes mellitus [1234]. Among them, Panax ginseng (Asian ginseng) and ginsam, a vinegar extract from P. ginseng, have been frequently reported to decrease blood glucose levels and body weights in animal models [567] and in humans [4]. Ginsenosides are biologically active components of P. ginseng, and there are many different types of ginsenoside with different pharmacological activities [8]. The ginsenoside Rg3, one of the most enriched components of ginsam, has been suggested as the major component producing anti-diabetic effects [6].
The main proposed mechanism of the anti-diabetic effect of P. ginseng is the improvement in insulin resistance in skeletal muscle rather than an increase in insulin secretion from pancreatic β-cells [56]. It has been demonstrated that Rg3 activates the phosphorylation of insulin receptor substrate-1 (IRS-1) and Akt, and glucose uptake in L6 myotubes [9].
Mitochondria play a pivotal role in the insulin resistance of skeletal muscle [1011]. Therefore, it is hypothesized that the improvement in insulin resistance by P. ginseng or Rg3 is, at least in part, mediated through the regulation of mitochondrial biogenesis or function in skeletal muscle. Here, we investigated the effects of Rg3 on the mitochondria of skeletal muscle using C2C12 myotubes.
C2C12 myoblasts (ATCC, Manassas, VA, USA) were maintained in Dulbecco's Modified Eagle Medium (DMEM) supplemented with 10% fetal bovine serum (Invitrogen, Carlsbad, CA, USA). Two days after the cells reached confluence, differentiation was induced with 1×high-glucose DMEM with 2% horse serum (Invitrogen) for 4 days. To induce insulin resistance, C2C12 myotubes were treated with 500 µM palmitate (Sigma-Aldrich Co., St. Louis, MO, USA) for 24 hours. To induce mitochondrial dysfunction, C2C12 myotubes were treated with 10 µM antimycin A (Sigma-Aldrich Co.) for 48 hours. After pretreatment with antimycin A, palmitate, or dimethyl sulfoxide (DMSO), 100 µM Rg3 (Sigma-Aldrich Co.) or DMSO was added to the medium for 24 hours. In some experiments, 10 µM compound C (Sigma-Aldrich Co.) was added 1 hour before Rg3 treatment.
Total RNA from C2C12 cells was isolated using TRIzol (Invitrogen). Reverse transcription polymerase chain reaction (RT-PCR) was performed using SYBR-master mix (TaKaRa, Kyoto, Japan) and the ABI 7500 RT-PCR system (Applied Biosystems, Foster City, CA, USA). The primer sequences for RT-PCR are shown in Table 1. All data were analyzed using ABI 7500 software version 2.0.5. (Applied Biosystems).
C2C12 cells were homogenized in 200 µL of mammalian cell lysis solution (ATPlite; PerkinElmer Inc., Waltham, MA, USA) using a micro-homogenizer. The cell lysates were aliquoted into a 96-well microplate (50 µL per well); 50 µL of substrate solution (ATPlite) was added to each well. After a dark adaptation for 10 minutes, luminescence was detected on a microplate reader (VICTOR3; PerkinElmer Inc.).
C2C12 cells were cultured in DMEM medium (Sigma-Aldrich Co.). The oxygen consumption rate (OCR) was measured using the Seahorse XF24 analyzer (Seahorse Bioscience Inc., North Billerica, MA, USA) according to the manufacturer's protocol. The OCR was measured under basal conditions and after the addition of the mitochondrial inhibitor oligomycin (2 µM) and the mitochondrial uncoupler carbonyl cyanide 4-(trifluoromethoxy)phenylhydrazone (FCCP; 5 µM) to assess maximal oxidative capacity. Antimycin A and rotenone were then added to inhibit the mitochondrial complex completely.
C2C12 cells were lysed in 20 mM Tris-HCl (pH 7.4), 5 mM Na4P2O7, 100 mM NaF, 2 mM Na3VO4, and 1% NP-40 buffer supplemented with a protease inhibitor (1 µg/µL aprotinin, 1 µg/µL leupeptin, and 1 mM PMSF). Cell lysates were sonicated on ice twice for 15 seconds each time, and debris was removed by centrifugation (15,000 g) for 30 minutes at 4℃. Proteins (10 to 20 µg) were separated on a sodium dodecyl sulfate-polyacrylamide gel and transferred to a nitrocellulose membrane (Whatman, Dassel, Germany). The membrane was blocked with 5% skim milk in Tween-20-Tris-buffered saline (TBS-T) for 1 hour at room temperature, followed by incubation with primary antibody overnight at 4℃. The primary antibodies used were a total OXPHOS antibody cocktail (MitoScience, San Francisco, CA, USA), anti-IRS-1 (Santa Cruz Biotechnology Inc., Santa Cruz, CA, USA), anti-pIRS-1 (Cell Signaling, Danvers, MA, USA), anti-Akt (Cell Signaling), anti-pAkt (Cell Signaling), anti-adenosine monophosphate (AMP)-activated protein kinase (AMPK; Cell Signaling), anti-phosphorylated AMPK (Cell Signaling), and anti-γ-tubulin (Sigma-Aldrich Co.). The secondary antibodies were anti-rabbit or anti-mouse immunoglobulin G.
Insulin stimulated IRS-1 and Akt phosphorylation in C2C12 myotubes, and Rg3 treatment for 24 hours enhanced the insulin signaling pathway (Fig. 1). To induce insulin resistance, we pretreated the cells with palmitate, and it decreased IRS-1 and Akt phosphorylation. Under that condition, Rg3 treatment increased IRS-1 and Akt phosphorylation (Fig. 1A) Even after pretreatment with antimycin A, the oxidative phosphorylation complex III inhibitor, Rg3 increased IRS-1 and Akt phosphorylation (Fig. 1B), suggesting that Rg3 can improve insulin signaling which has been deteriorated by mitochondrial dysfunction.
Next, we assessed the effects of Rg3 on mitochondrial function by measuring cellular adenosine triphosphate (ATP) levels or the OCR. Under basal conditions, there was no difference in cellular ATP levels after Rg3 treatment (Fig. 2A). However, after the induction of mitochondrial dysfunction by antimycin A, Rg3 significantly increased ATP production from 68% to 84% (P=0.03) (Fig. 2A). The effects of Rg3 on mitochondrial function were more pronounced when analyzing the OCR. The overall OCR after Rg3 treatment was increased (Fig. 2B). The OCR increase was clearly demonstrated under the conditions of antimycin A-induced mitochondrial dysfunction; the basal OCR was significantly increased by 2.8-fold (P=0.03) (Fig. 2C).
Next, we evaluated the quantitative changes in the protein or mRNA levels of mitochondrial complexes. Both Western blotting and quantitative RT-PCR analysis revealed that Rg3 increased CoxII of complex IV and F1α of complex V, while the effects of Rg3 were minimal or inconsistent in the other mitochondrial complexes (Fig. 3A and B). Under antimycin A-induced mitochondrial dysfunction, similar effects were observed. Therefore, we next determined the expression of transcription factors which regulate the expression of mitochondrial proteins, using quantitative RT-PCR. The expression of peroxisome proliferator-activated receptor γ coactivator 1α (PGC-1α), nuclear respiratory factor 1 (NRF-1), and mitochondrial transcription factor (Tfam) was significantly increased after Rg3 treatment, regardless of the presence of antimycin A (P<0.05) (Fig. 3C). These results suggest that Rg3 enhances expression of mitochondrial proteins through an increase of these transcription factors, which leads to the promotion of mitochondrial biogenesis.
AMPK is a major regulator of not only glucose and lipid metabolism but also mitochondrial biogenesis [121314]. The AMPK pathway has been proposed to be the mechanism of the improvement in insulin resistance by Rg3 in skeletal muscle [615]. Therefore, we examined whether AMPK mediated the effect of Rg3 on mitochondria using compound C, an AMPK inhibitor. However, compound C did not alter the expression of mitochondrial complexes (Fig. 4), suggesting that Rg3 does not regulate mitochondrial biogenesis through the AMPK pathway.
In this study, we first demonstrated that Rg3 improves mitochondrial function and the expression of key genes involved in mitochondrial biogenesis in C2C12 myotubes. This might be the mechanism underlying Rg3-enhanced insulin signaling in skeletal muscle. Our findings posit a possible mechanism behind the improvement in insulin sensitivity by P. ginseng or ginsam: the regulatory effects of Rg3 on skeletal muscle mitochondria may mediate the anti-diabetic effects of P. ginseng or ginsam, at least in part.
Because the majority of glucose uptake occurs in skeletal muscle, insulin sensitivity in skeletal muscle is important to maintain plasma glucose levels [16]. Insulin binding to the insulin receptor leads to the phosphorylation of IRS-1, which subsequently activates the phosphoinositide 3-kinase/Akt pathway and results in increased glucose uptake [17]. We previously reported that Rg3 increased insulin sensitivity by inducing IRS-1 and Akt phosphorylation, and the subsequent expression of GLUT4 and glucose uptake in skeletal muscle [69]. In this study, we reconfirmed the activation of IRS-1 and Akt by Rg3 in C2C12 cells, and to elucidate the underlying mechanism of Rg3, we focused on the mitochondria.
Mitochondria play an important role in the insulin resistance of skeletal muscle [1011]. Reduced mitochondrial numbers and impaired mitochondrial function have been observed in the skeletal muscle of diabetes mellitus patients [11]. Therefore, we investigated changes in the mitochondrial biogenesis and function after Rg3 treatment. We found that Rg3 significantly increased ATP production and the OCR, indicating an enhancement of mitochondrial function in skeletal muscle. These results are consistent with those of a previous study, which reported that Rg3 enhanced ATP production and the respiratory control ratio in the rat brain [18].
Mitochondrial dysfunction can also impair fatty acid oxidation and increase the production of reactive oxygen species (ROS), leading to the accumulation of intramyocellular fat content and ROS, and consequently, insulin resistance. Previous studies have reported that Rg3 stimulates the phosphorylation of acetyl-CoA carboxylase, a key regulator of fatty acid oxidation in C2C12 myotubes [15] and cardiac muscle [19]. Rg3 also increased antioxidant enzymes in rat cardiac muscle [19] and decreased ROS production in brain mitochondria [18]. Although we did not examine fatty acid oxidation and ROS production, Rg3 is expected to improve fatty acid oxidation and ROS production based on the previous reports.
We also demonstrated that Rg3 improved the expression of key genes involved in mitochondrial biogenesis. In this study, Rg3 treatment increased the expression of PGC-1α and its downstream targets, NRF-1 and Tfam. PGC-1α is a key regulator that induces mitochondrial biogenesis by activating other transcription factors, such as NRF-1 and NRF-2, which subsequently activate the expression of Tfam [20]. Previous studies have demonstrated that Rg3 increases the expression of PGC-1α in cardiac muscle [19] and skeletal muscle [615]. Following the change in the expression levels of transcription factors, we observed an increase in both the mRNA expression and protein levels of some components of the mitochondrial complexes in C2C12 cells. While Rg3 increased the expression of key genes involved in mitochondrial biogenesis regardless of the pretreatment of antimycin A (Fig. 2), Rg3 improved ATP production or the OCR only under the antimycin A-treated condition (Fig. 3). There are several reasons why Rg3 improved mitochondrial function against antimycin A but not under the basal condition. One possibility is that stimulation of the mitochondrial biogenesis could not preserve or potentiate mitochondrial function under the basal condition but could facilitate the recovery of mitochondrial function after mitochondrial inhibition [2122]. Another possibility is the difference in the post-translational regulation according to the conditions.
AMPK senses the energy status of the cell through the AMP/ATP ratio in the muscle, and a low energy status, such as that produced during exercise, activates AMPK and regulates energy metabolism, including glucose uptake and mitochondrial biogenesis [2324]. AMPK is also known to stimulate PGC-1α through the cAMP-response element binding protein (CREB), subsequently increasing mitochondrial biogenesis [202526]. It is possible that AMPK mediates the effect of Rg3 on mitochondrial biogenesis. Rg3 or ginsam has been reported to activate AMPK phosphorylation in C2C12 myotubes [15] and the skeletal muscle of OLETF rats [6]. However, in our previous study [9], Rg3 did not activate AMPK, and compound C did not alter insulin signaling in L6 myotubes. Consistent with previous findings, AMPK seems not to mediate mitochondrial biogenesis in this study (Fig. 4).
In conclusion, we demonstrated that Rg3, a constituent of P. ginseng, improves mitochondrial biogenesis and function, leading to the improvement in insulin resistance in skeletal muscle. Therefore, Rg3 may contribute to the anti-diabetic effect of P. ginseng, and it may be a potential therapeutic agent for the treatment of type 2 diabetes mellitus.
Figures and Tables
![]() | Fig. 1Effects of Rg3 on the insulin signaling pathway. In C2C12 myotubes, 100 µM Rg3 was administered for 24 hours with or without (A) 500 µM palmitate or (B) 10 µM antimycin A. Experiment was repeated four times. pIRS-1, phosphorylated insulin receptor substrate-1; IRS-1, insulin receptor substrate-1. |
![]() | Fig. 2Effects of Rg3 on mitochondrial function. In C2C12 myotubes, 100 µM Rg3 was administered for 24 hours with or without 10 µM antimycin A. (A) Cellular adenosine triphosphate (ATP) levels. (B) Representative graphs of oxygen consumption rate (OCR). OCR was measured under the basal conditions, following the addition of oligomycin, carbonyl cyanide 4-(trifluoromethoxy)phenylhydrazone (FCCP), and antimycin A/rotenone. (C) Basal OCR. Each experiment was repeated more than four times. Data was presented as mean±standard error of mean. aP<0.05. |
![]() | Fig. 3Effects of Rg3 on the expression of key genes involved in mitochondrial biogenesis. In C2C12 myotubes, 100 µM Rg3 was administered for 24 hours with or without 10 µM antimycin A. (A) Expression of mitochondrial complexes by Western blot. (B) mRNA expression of mitochondrial complexes by quantitative reverse transcription polymerase chain reaction (RT-PCR). (C) mRNA expression of peroxisome proliferator-activated receptor γ coactivator 1α (PGC-1α), nuclear respiratory factor 1 (NRF-1), and mitochondrial transcription factor (Tfam) by quantitative RT-PCR. RT-PCR was performed in duplicate, and each experiment was repeated five times. aP<0.05. |
![]() | Fig. 4Effects of Rg3 and adenosine monophosphate (AMP)-activated protein kinase (AMPK) pathway. After pretreatment of 10 µM antimycin A, 100 µM Rg3 was administered for 24 hours with or without 10 µM compound C in C2C12 myotubes. The expression of mitochondrial complexes and AMPK was evaluated by Western blot. pAMPK, phosphorylated AMPK. |
Table 1
Primer sequences used for quantitative reverse transcription polymerase chain reaction
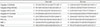
ACKNOWLEDGMENTS
This study was supported by grants by the Ministry of Science and Technology (M1064214004-06N4214-00410).
References
1. Vuksan V, Sievenpiper JL, Koo VY, Francis T, Beljan-Zdravkovic U, Xu Z, Vidgen E. American ginseng (Panax quinquefolius L) reduces postprandial glycemia in nondiabetic subjects and subjects with type 2 diabetes mellitus. Arch Intern Med. 2000; 160:1009–1013.
2. Vuksan V, Stavro MP, Sievenpiper JL, Beljan-Zdravkovic U, Leiter LA, Josse RG, Xu Z. Similar postprandial glycemic reductions with escalation of dose and administration time of American ginseng in type 2 diabetes. Diabetes Care. 2000; 23:1221–1226.
3. De Souza LR, Jenkins AL, Sievenpiper JL, Jovanovski E, Rahelic D, Vuksan V. Korean red ginseng (Panax ginseng C.A. Meyer) root fractions: differential effects on postprandial glycemia in healthy individuals. J Ethnopharmacol. 2011; 137:245–250.
4. Yoon JW, Kang SM, Vassy JL, Shin H, Lee YH, Ahn HY, Choi SH, Park KS, Jang HC, Lim S. Efficacy and safety of ginsam, a vinegar extract from Panax ginseng, in type 2 diabetic patients: results of a double-blind, placebo-controlled study. J Diabetes Investig. 2012; 3:309–317.
5. Attele AS, Zhou YP, Xie JT, Wu JA, Zhang L, Dey L, Pugh W, Rue PA, Polonsky KS, Yuan CS. Antidiabetic effects of Panax ginseng berry extract and the identification of an effective component. Diabetes. 2002; 51:1851–1858.
6. Lim S, Yoon JW, Choi SH, Cho BJ, Kim JT, Chang HS, Park HS, Park KS, Lee HK, Kim YB, Jang HC. Effect of ginsam, a vinegar extract from Panax ginseng, on body weight and glucose homeostasis in an obese insulin-resistant rat model. Metabolism. 2009; 58:8–15.
7. Yun SN, Ko SK, Lee KH, Chung SH. Vinegar-processed ginseng radix improves metabolic syndrome induced by a high fat diet in ICR mice. Arch Pharm Res. 2007; 30:587–595.
8. Attele AS, Wu JA, Yuan CS. Ginseng pharmacology: multiple constituents and multiple actions. Biochem Pharmacol. 1999; 58:1685–1693.
9. Kim M, Ahn BY, Lee JS, Chung SS, Lim S, Park SG, Jung HS, Lee HK, Park KS. The ginsenoside Rg3 has a stimulatory effect on insulin signaling in L6 myotubes. Biochem Biophys Res Commun. 2009; 389:70–73.
10. Kelly SP, Wood CM. Prolactin effects on cultured pavement cell epithelia and pavement cell plus mitochondria-rich cell epithelia from freshwater rainbow trout gills. Gen Comp Endocrinol. 2002; 128:44–56.
11. Ritov VB, Menshikova EV, He J, Ferrell RE, Goodpaster BH, Kelley DE. Deficiency of subsarcolemmal mitochondria in obesity and type 2 diabetes. Diabetes. 2005; 54:8–14.
12. Kahn BB, Alquier T, Carling D, Hardie DG. AMP-activated protein kinase: ancient energy gauge provides clues to modern understanding of metabolism. Cell Metab. 2005; 1:15–25.
13. Hardie DG. AMP-activated protein kinase: a key system mediating metabolic responses to exercise. Med Sci Sports Exerc. 2004; 36:28–34.
14. Hardie DG, Sakamoto K. AMPK: a key sensor of fuel and energy status in skeletal muscle. . Physiology (Bethesda). 2006; 21:48–60.
15. Park MW, Ha J, Chung SH. 20(S)-ginsenoside Rg3 enhances glucose-stimulated insulin secretion and activates AMPK. Biol Pharm Bull. 2008; 31:748–751.
16. DeFronzo RA, Gunnarsson R, Bjorkman O, Olsson M, Wahren J. Effects of insulin on peripheral and splanchnic glucose metabolism in noninsulin-dependent (type II) diabetes mellitus. J Clin Invest. 1985; 76:149–155.
17. Saltiel AR, Kahn CR. Insulin signalling and the regulation of glucose and lipid metabolism. Nature. 2001; 414:799–806.
18. Tian J, Zhang S, Li G, Liu Z, Xu B. 20(S)-ginsenoside Rg3, a neuroprotective agent, inhibits mitochondrial permeability transition pores in rat brain. Phytother Res. 2009; 23:486–491.
19. Sun M, Huang C, Wang C, Zheng J, Zhang P, Xu Y, Chen H, Shen W. Ginsenoside Rg3 improves cardiac mitochondrial population quality: mimetic exercise training. Biochem Biophys Res Commun. 2013; 441:169–174.
20. Hock MB, Kralli A. Transcriptional control of mitochondrial biogenesis and function. Annu Rev Physiol. 2009; 71:177–203.
21. Rasbach KA, Schnellmann RG. PGC-1alpha over-expression promotes recovery from mitochondrial dysfunction and cell injury. Biochem Biophys Res Commun. 2007; 355:734–739.
22. Chowanadisai W, Bauerly KA, Tchaparian E, Wong A, Cortopassi GA, Rucker RB. Pyrroloquinoline quinone stimulates mitochondrial biogenesis through cAMP response element-binding protein phosphorylation and increased PGC-1alpha expression. J Biol Chem. 2010; 285:142–152.
23. O'Neill HM. AMPK and exercise: glucose uptake and insulin sensitivity. Diabetes Metab J. 2013; 37:1–21.
24. Jorgensen SB, Wojtaszewski JF, Viollet B, Andreelli F, Birk JB, Hellsten Y, Schjerling P, Vaulont S, Neufer PD, Richter EA, Pilegaard H. Effects of alpha-AMPK knockout on exercise-induced gene activation in mouse skeletal muscle. FASEB J. 2005; 19:1146–1148.
25. Jager S, Handschin C, St-Pierre J, Spiegelman BM. AMP-activated protein kinase (AMPK) action in skeletal muscle via direct phosphorylation of PGC-1alpha. Proc Natl Acad Sci U S A. 2007; 104:12017–12022.
26. Thomson DM, Herway ST, Fillmore N, Kim H, Brown JD, Barrow JR, Winder WW. AMP-activated protein kinase phosphorylates transcription factors of the CREB family. . J Appl Physiol (1985). 2008; 104:429–438.