Abstract
Objective
We describe a technique to induce in vitro traumatic brain injury in an organotypic slice culture (OTC).
Methods
Centrifugal forces were applied to rat hippocampal slices at strengths ranging from 300 to 7,500 g.
Results
The degree of neural injury correlated with the forces applied. A morphometric analysis of hippocampal CA area revealed significant tissue expansion. A graded posttraumatic decrease in evoked field potentials was recorded from acutely prepared slices. Immediate posttraumatic cell death was identified at the base of the slice where it was attached to the porous membrane. Delayed cell death and secondary damage induced by serum deprivation, excitotoxicity, hypoxic insults, and additional impacts were investigated and observed by conventional fluorescent microscopy.
Several in vitro models focusing on cells or tissues have been described and used to induce traumatic brain injuries (TBI). These models include the effects of transaction,13,29,37) compression,4) acceleration,24) hydrostatic pressure,38) hydrodynamic forces21) and stretch.11) In comparison with cultured cells, cultured or sliced organotypic brain tissues contain heterogeneous populations of cells and have the advantage of maintaining complex three-dimensional connections. Acute hippocampal slices (AHSs) have a long history of successful application in neural science investigations, and the organotypic slice culture (OTC), cultured on semiporous membranes, has become popular for studying mechanisms of brain damage. Because these fragments of nerve tissue are very small, it is not easy to induce reproducible, targeted damage. Previously described damage methods have, therefore, been limited to weight drop,1,4,33) barotraumas38) and substrate strain.27,28)
The centrifuge is commonly used in laboratories to apply a known amount of centrifugal force to target tissues. Tissues under acceleration undergo an increase in pressure, and this generates strain and transformation. Thus, centrifugal forces applied to tissues provide a reliable in vitro model of static compression injury, producing reproducible damage without special equipment. We have investigated the benefits of this model.
Male Sprague Dawley rats, 4-6 weeks of age and weighing 100-150 g, were anesthetized with 3% halothane and decapitated. The brains were rapidly removed and placed in artificial cerebrospinal fluid (aCSF), chilled to 0-4℃ and oxygenated with a mixture of 95% O2 and 5% CO2. The hippocampi were removed from each rat brain and cut into 450 µm thick transverse slices using a McIlwain tissue chopper. Slices were incubated in vials of continually oxygenated aCSF at 30±1℃ before use. The aCSF consisted of 124 mM NaCl, 3.0 mM KCl, 2.0 mM CaCl2, 2.0 mM MgCl2, 1.2 mM NaH2PO4, 26 mM NaHCO3 and 10 mM d-glucose. Aeration with 5% CO2 in O2 resulted in an aCSF pH of 7.4 ± 0.05. After a 90 min recovery period, each slice was laid on a piece of lens cleansing paper (Cat. No. 2105 862, Whatman) for later handling using forceps (Figure 1). A blind protocol was used to assign slices to either a sham-injured or injury group, and for analyzing the results. All animal procedures were reviewed and approved by the Korea University Institutional Animal Care and Use Committee, and complied with guidelines outlined in the Korean Academy of Medical Science's Guide for the Care and Use of Laboratory Animals.
Seven-day-old Sprague Dawley rat pups were anesthetized with halothane and plunged into a 70% alcohol solution. They were decapitated, and transverse hippocampal slices (400 µm) were taken and kept in an ice-cold stabilization medium for approximately 30 min. The medium contained 50% minimal essential medium (MEM) with no bicarbonate or glutamate, 50% calcium- and magnesium-free Hanks' balanced salt solution (HBSS), 7.5 mM glucose and 20 mM N-2-hydroxyethyl piperazine-N'-2-ethanesulfonic acid (HEPES), at pH 7.15. Following stabilization, slices were separated and transferred to 25 mm inserts, each containing a porous (0.4 µm) Millicel-CM sterile tissue culture membrane (Millipore, Bedford, MA, USA). Inserts, each with six equally spaced hippocampal tissue slices, were transferred to six-well culture trays with 1 mL of growth medium in each well. The medium consisted of 50% MEM, 25% horse serum, 25% Earl's balanced salt solution with glucose and HEPES, 5,000 units/mL penicillin G and 50 mg/mL streptomycin sulfate, adjusted to pH 7.3. Slices were cultured for 14 days at 36.5℃ and 100% humidity, in an atmosphere of 95% air and 5% CO2 and fed twice weekly by 50% medium exchange. To minimize culture variability, each set of control and experimental OTCs within a study were cultured on the same day. The inserts were blindly assigned to either a control, sham-injured or injury group; each plate also contained a control insert representing the status of the plate under normal culture conditions during the period of the experiment.
We used two different methods to apply centrifugal stress. OTCs on their 25 mm inserts were simply placed at the bottom of a sterile centrifuge bucket in a swinging rotor. This type of centrifuge has a slightly longer period of acceleration, with experimental forces lasting up to 3 min (including the acceleration and plateau phases). For AHSs we used an angled rotor with a rapid acceleration phase. To arrange AHSs perpendicular to the axis of rotation in the angled rotor, melted paraffin was poured into an Eppendorff tube and the flat paraffin surface so formed was positioned parallel to the rotational axis (Figure 1). Sham-injured slices were subjected to all procedures except centrifugation.
Fluorescence microscopy was used to analyze results from some AHS experiments. First, the nuclei in the pyramidal layer at the slice surface killed during slice preparation were labeled by cultivating the slices in aCSF containing 10 µg/mL propidium iodide (PI) for 30 min prior to injury. The slices were photographed at 40× magnification before injury and again under the same conditions after centrifugal acceleration. The area of the cornus ammoni (CA) field between the hilus and CA1 was measured using image analyzing software before and after injury, and the extent of any change expressed as a percentage.
After a 30 min recovery period, the AHSs backed by the lens paper were transferred to a submerged 0.12 mL recording chamber. The superfusing aCSF (2 mL/min) was continually aerated and its temperature was kept at 32-33℃. Evoked extracellular population spikes (PSs) were recorded in the stratum pyramidale of CA1 following electrical stimulation of the Schaffer collateral commissural pathway. The spikes were recorded with glass micropipettes filled with 150 mM NaCl (2-5 MΩ). The field potentials were amplified, filtered and recorded on-line with a computer interface. Stimuli (50 µs in duration) were delivered at 0.05 Hz through bipolar platinum-iridium electrodes with tip diameters of 25 µm, placed in the stratum radiatum. The minimum strength necessary to elicit a maximal orthodromic population spike (PS) was applied and the PS observed for at least 30 min.
Viability and cell death in OTCs was examined by the exclusion of fluorescent dye using an inverted fluorescent microscope after culturing the slices in a medium containing 7.5 µM PI. Cultures displaying a high initial PI uptake were excluded from further experiments. The cell death index derived from the fluorescent intensity measure was normalized to the values obtained after the slices were exposed to an ambient temperature of 4℃, which results in maximal cell death. Pixel intensity within a selected area was averaged to enable statistical comparisons to be made. Some OTCs were exposed to a secondary injury between 12 and 24 hr after the initial injury. Hypoxic injury was applied by placing OTCs in a 37℃ incubator gassed with 95% N2/5% CO2. Exposing OTCs to 10 mM glutamate, or depriving them of serum, induced excitotoxic and apoptotic cell death. A second impact was delivered by repeating the centrifugation at an identical strength. All slices from the same plate were discarded from the study if OTCs in the control insert showed more than slight PI (-5%) fluorescence at the end of the experiment. All recorded data were expressed as mean±standard errors. Two sample t-tests were carried out for the comparisons between the groups. The results with p values less than 0.05 were considered to be significantly different.
The morphological changes in acute hippocampal slices after centrifugal acceleration are characterized by flattening of slices, expansions of subfields, splits in natural fissures, cleavages of cellular layers, and loss of tissue (Figure 2A). The transformation was particularly severe within the dentate gyrus (DG), with the disruption of tissue making morphometric assessment of the degree of expansion unreliable. The field expansions were distinctive even in the hilar region. In CA regions the slices retained their original morphology well, with centrifugal forces applied to an AHS for 60 sec showing a graded effect on tissue expansion (Figure 2B).
The PS amplitude measured 30 min following sham injury (at 1 g) on the control slice was 6.6+/-0.5 mV. Increasing the force for 60 s gradually reduced the amplitudes of PS with the minimum level (<10%) being recorded at 5,000 g (Figure 3A). We arbitrarily divided the force applied for sham injury to minimum level into five levels to obtain a graded injury (500, 1,500, 2,300, 3,000, and 5,000 g). The mean PS amplitudes measured at 30 min after injury were significantly different between these levels. At 2,300 g, a 20 s step increase in duration also revealed statistical differences in PS values (Figure 3B).
Four days of posttraumatic observations revealed that the peak PI uptake developed within the first 24 hr after trauma, and slightly decreased thereafter (Figure 4). During the observation period, cell death in sham injured cultures was minimal. The most unexpected observation was a widespread increase in PI uptake of about 25% seen at 1 hr in the OTCs subjected to either slight (300 g) or moderate (3,000 g) centrifugation. Twelve hours after injury, diffuse PI uptake was less well defined and delayed degeneration began in the neuronal layers, usually in the inner blade of the dentate gyrus (Figure 5). Three different strengths of centrifugal force (300 g, 1,000 g, and 3,000 g) resulted in graded peak uptakes of PI. Cell death was prominent in both the dentate gyrus (DG) and CA1 in more severely damaged OTCs, but the progression of cell necrosis was minimal up to the end of the experiment, regardless of the force intensity. With forces stronger than 5,000 g, OTCs frequently became detached from the porous membrane, making measurements of identical areas impossible. Secondary exposure to excitotoxicity (glutamate, 10 mM for 30 min, n=12), or hypoxia (for 60 min, n=12) led to increased cell death in the neuronal layers of both sham-injured and injured OTCs, whereas serum deprivation (for 24 hr, n=12) or additional impact (300 g, n=12) was more likely to produce diffuse patterns of cell death in traumatized OTCs (Figure 6).
Static compression of nervous tissue has been a classical model of traumatic injury for many years. One of its limitations is that the mechanism of dynamic impact is not included. Nevertheless, by adjusting both the extent and duration of compression, controlled, graded injuries can be produced. In vivo spinal cord models include clip compression30,31) and subdural balloon inflation,25) with acute subdural compression7,20) used for the intact brain. Clip compression was used in vitro on a strip of dorsal column from the spinal cord.14) The cylindrical shape of optic nerves and spinal cord strips facilitates the application of calibrated compressions. In contrast, thin, wide brain slices require more delicate and sophisticated techniques because they lack supportive structures. Limited access time without perfusion is another potential problem with in vitro experiments: only a few minutes spent adjusting the apparatus and sample tissue can destroy the tissue. The technique described here, however, does not suffer from these limitations, and in addition provides easy, reproducible adjustment of the applied force. Special training is not required, which minimizes variations in the injury applied. The major advantage of this injury system is its versatility. A single apparatus suitable for producing injury in both acute preparations and OTCs has not been described previously. Although we have only tested it on hippocampal slices, it should work well with other tissue preparations. The force applied to the tissue with this technique is simply expressed in gravitation units, making direct comparisons with data from other experiments possible.
The effect of acceleration on the brain depends on its duration and strength, and on the brain's biophysical properties. Studies performed in the 1950s that exposed human volunteers to up to 40 g of sagittal deceleration in a linear decelerator did not produce lasting neurological complaints or evidence of permanent brain damage.36) However, blunt trauma sustained in falls from standing positions can generate 100 g of force, and produce fatal head injuries.16) The brain generally is better able to tolerate slow increases in acceleration, even though the total amount of strain is identical. Wearing a helmet increases the duration of impact and lowers the peak force, as shown by accelerometer measurements.22) Helmeted racing car drivers have had no brain injuries from accidents involving head decelerations of over 200 g, and jet aircraft test pilots have been subjected to up to 450 g without concussion.34) Small tissue slices offer several advantages over whole human brains in the study of mechanical injury. The ratio of brain weight to surface area is low in small brain and tissue samples, and therefore a given impact deceleration will be spread over a correspondingly greater area. The acceleration required to produce injury in brains with similar properties and shapes should be inversely proportional to the two-thirds power of their mass. The acceleration required to produce an injury similar in extent in whole human would, therefore, need to be 400-500 times greater for 450 µm slices. The functional loss in the human brain assumed to be caused by a dynamic impact of 60 g would require up to 30,000 g in brain slices. Small size is one reason why woodpecker brains, weighing 1.25-3.95 g, can withstand repeated impact forces of the order of 1,000 g during impact drilling.26) The single impact acceleration threshold to produce concussion in dogs is between 250 g and 500 g.18) Even with same acceleration applied in a well-designed centrifugal system, tissue transformation is affected by other physical conditions. One variable is the density and elasticity of the slices, especially in AHS preparations, which are affected by temperature and incubation time during preparation and storage. Tissue slices gradually become swollen due to surface autolysis and water uptake increasing the damage; this begins within minutes of preparation.5) Testing the slices at the same temperatures and incubation duration can minimize this damage. Even with identical tissue densities and elasticities, the pressure effects vary within the thickness of the slice. Generally, structures in the middle are not deformed by ambient gravity. However, the increased pressure from overlying tissue is multiplied by the g force such that, in thicker slices, the deeper regions are more deformed by centrifugation. Therefore, measurement of tissue at the same depth from the top surface is a key to obtaining reproducible results. For this reason, the AHS data (usually recorded 150 µm from the surface) are more consistent than images from OTCs obtained from the bottom of the slices. Additional injury caused by the stretching of the suspended porous membrane in the inserts during centrifugation might also be responsible for the observed variation. This membrane is elastic and is attached at the wall, just above the bottom of the insert. It is at first stretched downwards during centrifugation until it comes into contact with the floor of the apparatus. Such initial, limited strain and shear between the slices and the stretched membrane may be responsible for the widespread uniform intensity in PI uptake measured at 1 hr, regardless of the force applied.
The prominent injuries observed in this study, especially in the medial blade of the dentate gyrus in both AHSs and OTCs, are striking. Although all areas of the slice are equally exposed to the force in this experimental technique, the circular configuration of the cell layers in the dentate gyrus may offer a localized area of relative weakness to shear forces. In vivo studies have reported obvious differences in the pattern of cell death in various models.15) Although studies using cortical impact or fluid percussion injury have demonstrated significant neuronal cell loss in the CA2/CA3 and hilar regions of the dentate gyrus3,17,35) other investigators have noted dentate hilar cell loss with no significant loss in CA1, CA2, or CA3 using the same paradigm.6,23) Neuronal loss in the dentate gyrus is confined to the hilus and delayed in onset, but it is probable that dentate granule cells showing early32) and delayed23) posttraumatic hyperexcitability are also susceptible to trauma. Similarly, the pyramidal cells in CA1 with posthypoxic hyperexcitability10) have been shown to be selectively vulnerable to hypoxia.2,19) Although there is no evidence, perhaps the early, five-fold increase in the number of progenitor cells in the dentate gyrus12) plays a role in replacing neurons lost in CA3, and possibly in the dentate gyrus itself. We also note the difference in the temporal pattern of posttraumatic cell death from previous models1,9) in which cell death is greatest at 3-4 days. We have no explanation for these differences, but our findings agree with previous in vivo studies in which neuronal loss reached a maximum in the first day post trauma, and remained near that level subsequently.3,8) The known selective vulnerability to hypoxia in CA1 and excitotoxicity in CA1 and CA3 was reproduced in our trauma model. However, secondary impact or apoptosis induced by serum deprivation produced a more widespread cell death in our study, suggesting that these insults might have a tendency to kill glia as well as neurons in the posttraumatic state.
In conclusion, we showed novel compressive model of neural injury. It may be useful for neurotrauma research because it is accessible, easily adjustable, and reliable.
Figures and Tables
FIGURE 1
Diagram of the apparatus used to induce centrifugal acceleration injury on acute hippocampal slices (AHSs), with a representative photomicrograph of an AHS. The floor of the Eppendorff tube is shaped by pouring melted paraffin (P) into it so that it flows parallel to the axis of rotation. The centrifugal force (arrow) acts perpendicularly to the slice.
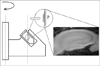
FIGURE 2
Morphometric analyses of the CA field areas of acute hippocampal slices after centrifugal injuries. A: Examples of propidium iodide labeling (10 µg/mL) of slices before and after 1,500-7,500 g centrifugal force. B: A graph showing changes in CA field area after graded injuries. The results are presented as the mean±SE. Numbers indicate number of experiments. Asterisks indicate a significant difference between groups (p<0.05).
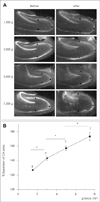
FIGURE 3
Evoked potential recordings in AHSs. A: Graph showing the amplitude of the maximally evoked orthodromic population spike in relation to the injury severity (60 s force application). B: Graph illustrating the relationship between the amplitude of maximally evoked orthodromic population spike and the duration of exposure at 2,300 g. Numbers indicate number of experiments. Asterisks indicate significant difference between groups (p<0.05). AHSs: acute hippocampal slices.
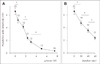
FIGURE 4
Cell death in OTCs induced by a centrifugal force of 300 g (marked by an arrow). A: Digital images of PI fluorescence in OTCs made before and for four days after injury, and at the final state of confirmed death. B: Summary graph illustrating cell death (as a percentage of the maximal level) in OTCs subjected to injury. The fluorescence emission of PI was measured over the whole hippocampal slice. Note that the peak PI uptake was developed within 24 hr of the injury. OTCs: organotypic slice culture, PI: propidium iodide.
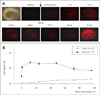
FIGURE 5
Cell death in OTCs subjected to centrifugal injury. A: Representative digital images of PI fluorescence in OTCs. Widespread PI uptake was observed at 1 hr in OTCs subjected to both light (300 g) and moderate (3,000 g) centrifugal forces, at a similar intensity. Twelve hours following injury, diffuse PI uptake became indistinct and delayed degeneration began in the neuronal layers, commonly in the inner blade of the dentate gyrus and CA1. B: Summary graph illustrating cell death measured in the whole hippocampal slice. C: Summary graph showing regional differences in cell death in OTCs measured 12 hr after injury. OTCs: organotypic slice culture, PI: propidium iodide, CA: cornus ammoni, DG: dentate gyrus.
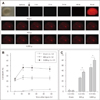
FIGURE 6
Representative digital images of PI fluorescence in traumatized OTCs (300 g) followed by an additional insult delivered between 12 and 24 hr later. Secondary exposure to (A) hypoxia (for 60 min) or (B) excitotoxicity (glutamate, 10 mM for 30 min) led to increased cell death in the neuronal layer both in sham-injured and injured OTCs. Secondary exposure to (C) serum deprivation (for 24 hr) or (D) additional impact (300 g) was more likely to produce diffuse patterns of cell death in traumatized OTCs. PI: propidium iodide, OTCs: organotypic slice culture.

References
1. Adamchik Y, Frantseva MV, Weisspapir M, Carlen PL, Perez Velazquez JL. Methods to induce primary and secondary traumatic damage in organotypic hippocampal slice cultures. Brain Res Brain Res Protoc. 2000; 5:153–158.


2. Aitken PG, Schiff SJ. Selective neuronal vulnerability to hypoxia in vitro. Neurosci Lett. 1986; 67:92–96.
3. Baldwin SA, Gibson T, Callihan CT, Sullivan PG, Palmer E, Scheff SW. Neuronal cell loss in the CA3 subfield of the hippocampus following cortical contusion utilizing the optical disector method for cell counting. J Neurotrauma. 1997; 14:385–398.


4. Balentine JD, Greene WB, Bornstein M. In vitro spinal cord trauma. Lab Invest. 1988; 58:93–99.
5. Bourke RS, Tower DB. Fluid compartmentation and electrolytes of cat cerebral cortex in vitro. I. Swelling and solute distribution in mature cerebral cortex. J Neurochem. 1966; 13:1071–1097.
6. Bramlett HM, Dietrich WD, Green EJ, Busto R. Chronic histopathological consequences of fluid-percussion brain injury in rats: effects of post-traumatic hypothermia. Acta Neuropathol. 1997; 93:190–199.


7. Bullock R, Butcher S, McCulloch J. Changes in extracellular glutamate concentration after acute subdural haematoma in the rat--evidence for an "excitotoxic" mechanism? Acta Neurochir Suppl (Wien). 1990; 51:274–276.
8. Carbonell WS, Grady MS. Evidence disputing the importance of excitotoxicity in hippocampal neuron death after experimental traumatic brain injury. Ann N Y Acad Sci. 1999; 890:287–298.


9. Cater HL, Sundstrom LE, Morrison B 3rd. Temporal development of hippocampal cell death is dependent on tissue strain but not strain rate. J Biomech. 2006; 39:2810–2818.


10. Doolette DJ, Kerr DI. Hyperexcitability in CA1 of the rat hippocampal slice following hypoxia or adenosine. Brain Res. 1995; 677:127–137.


11. Ellis EF, McKinney JS, Willoughby KA, Liang S, Povlishock JT. A new model for rapid stretch-induced injury of cells in culture: characterization of the model using astrocytes. J Neurotrauma. 1995; 12:325–339.


12. Emery DL, Fulp CT, Saatman KE, Schütz C, Neugebauer E, McIntosh TK. Newly born granule cells in the dentate gyrus rapidly extend axons into the hippocampal CA3 region following experimental brain injury. J Neurotrauma. 2005; 22:978–988.


13. Fayaz I, Tator CH. Modeling axonal injury in vitro: injury and regeneration following acute neuritic trauma. J Neurosci Methods. 2000; 102:69–79.


14. Fehlings MG, Nashmi R. Assessment of axonal dysfunction in an in vitro model of acute compressive injury to adult rat spinal cord axons. Brain Res. 1995; 677:291–299.


15. Floyd CL, Golden KM, Black RT, Hamm RJ, Lyeth BG. Craniectomy position affects morris water maze performance and hippocampal cell loss after parasagittal fluid percussion. J Neurotrauma. 2002; 19:303–316.


16. Foust DR, Bowan BM, Snyder RG. Study of human impact tolerance using investigations and simulations of free-fall. Society Automotive Engineers technical paper no. 770915. Prodeedings of 21 Stapp Car Crash Conference: 1976.
17. Goodman JC, Cherian L, Bryan RM Jr, Robertson CS. Lateral cortical impact injury in rats: pathologic effects of varying cortical compression and impact velocity. J Neurotrauma. 1994; 11:587–597.


18. Gurdjian ES, Roberts VL, Thomas LM. Tolerance curves of acceleration and intracranial pressure and protective index in experimental head injury. J Trauma. 1966; 6:600–604.


19. Kirino T, Sano K. Selective vulnerability in the gerbil hippocampus following transient ischemia. Acta Neuropathol. 1984; 62:201–208.


20. Kwon TH, Sun D, Daugherty WP, Spiess BD, Bullock MR. Effect of perfluorocarbons on brain oxygenation and ischemic damage in an acute subdural hematoma model in rats. J Neurosurg. 2005; 103:724–730.


21. LaPlaca MC, Thibault LE. An in vitro traumatic injury model to examine the response of neurons to a hydrodynamically-induced deformation. Ann Biomed Eng. 1997; 25:665–677.
22. Lewis LM, Naunheim R, Standeven J, Lauryssen C, Richter C, Jeffords B. Do football helmets reduce acceleration of impact in blunt head injuries? Acad Emerg Med. 2001; 8:604–609.


23. Lowenstein DH, Thomas MJ, Smith DH, McIntosh TK. Selective vulnerability of dentate hilar neurons following traumatic brain injury: a potential mechanistic link between head trauma and disorders of the hippocampus. J Neurosci. 1992; 12:4846–4853.


24. Lucas JH, Wolf A. In vitro studies of multiple impact injury to mammalian CNS neurons: prevention of perikaryal damage and death by ketamine. Brain Res. 1991; 543:181–193.


25. Martin D, Schoenen J, Delrée P, Gilson V, Rogister B, Leprince P, et al. Experimental acute traumatic injury of the adult rat spinal cord by a subdural inflatable balloon: methodology, behavioral analysis, and histopathology. J Neurosci Res. 1992; 32:539–550.


26. May PR, Fuster JM, Haber J, Hirschman A. Woodpecker drilling behavior. An endorsement of the rotational theory of impact brain injury. Arch Neurol. 1979; 36:370–373.
27. Morrison B 3rd, Cater HL, Benham CD, Sundstrom LE. An in vitro model of traumatic brain injury utilising two-dimensional stretch of organotypic hippocampal slice cultures. J Neurosci Methods. 2006; 150:192–201.


28. Morrison B 3rd, Meaney DF, McIntosh TK. Mechanical characterization of an in vitro device designed to quantitatively injure living brain tissue. Ann Biomed Eng. 1998; 26:381–390.
29. Mukhin AG, Ivanova SA, Knoblach SM, Faden AI. New in vitro model of traumatic neuronal injury: evaluation of secondary injury and glutamate receptor-mediated neurotoxicity. J Neurotrauma. 1997; 14:651–663.
30. Park YK, Tator CH. Failure of topical DMSO to improve blood flow or evoked potentials in rat spinal cord injury. J Korean Med Sci. 1998; 13:638–644.


31. Rivlin AS, Tator CH. Effect of duration of acute spinal cord compression in a new acute cord injury model in the rat. Surg Neurol. 1978; 10:38–43.
32. Santhakumar V, Bender R, Frotscher M, Ross ST, Hollrigel GS, Toth Z, et al. Granule cell hyperexcitability in the early post-traumatic rat dentate gyrus: the 'irritable mossy cell' hypothesis. J Physiol. 2000; 524(Pt 1):117–134.


33. Sieg F, Wahle P, Pape HC. Cellular reactivity to mechanical axonal injury in an organotypic in vitro model of neurotrauma. J Neurotrauma. 1999; 16:1197–1213.
34. Snively GG, Chichester CO. Impact survival levels of head acceleration in man. Aerosp Med. 1961; 32:316–320.
35. Soares HD, Sinson GP, McIntosh TK. Fetal hippocampal transplants attenuate CA3 pyramidal cell death resulting from fluid percussion brain injury in the rat. J Neurotrauma. 1995; 12:1059–1067.


36. Sweeney HM. Human decelerator. J Aviat Med. 1951; 22:39–41.