Abstract
Purpose
The aim of this study was to evaluate the surface properties and biological response of an anodized titanium surface by cell proliferation and alkaline phosphatase activity analysis.
Methods
Commercial pure titanium (Ti) disks were prepared. The samples were divided into an untreated machined Ti group and anodized Ti group. The anodization of cp-Ti was formed using a constant voltage of 270 V for 60 seconds. The surface properties were evaluated using scanning electron microscopy, X-ray photoelectron spectroscopy, and an image analyzing microscope. The surface roughness was evaluated by atomic force microscopy and a profilometer. The contact angle and surface energy were analyzed. Cell adhesion, cell proliferation, and alkaline phosphatase activity were evaluated using mouse MC3T3-E1 cells.
Results
The anodized Ti group had a more porous and thicker layer on its surface. The surface roughness of the two groups measured by the profilometer showed no significant difference (P>0.001). The anodized Ti dioxide (TiO2) surface exhibited better corrosion resistance and showed a significantly lower contact angle than the machined Ti surface (P>0.001). Although there was no significant difference in the cell viability between the two groups (P>0.001), the anodized TiO2 surface showed significantly enhanced alkaline phosphatase activity (P<0.001).
Titanium (Ti) alloys are widely used as biomaterials, such as in orthopedic and dental implants, because of their excellent mechanical strength, chemical stability, biocompatibility, and facilitated osseointegration [1]. The biocompatibility of Ti is closely related to its surface characteristics such as its chemical composition, surface roughness, and surface energy [2-5]. As a result, many attempts have been made to improve the surface properties of Ti implants and consequently to enhance initial bone bonding [6-10].
These surface modification techniques include sand blasting, acid etching, and plasma spraying. However, mechanical methods may be uncontrollable and imprecise, and form contaminant particles on Ti implant surfaces [1]. Similarly, acid etching has no ability to produce controllable surface topographies, and it also has the potential to form residual surface acids detrimental to bone growth [11]. Furthermore, the pore size of the implant surface formed through these processes is nonuniform at the microscale or macroscale. However, Sato and Webster [12] found that osteoblasts may be accustomed to a nanoscale rather than a microscale environment. Therefore, more desirable methods to modify Ti surfaces are needed for promoting bone growth.
The surface of Ti reacts with oxygen in the air and spontaneously forms a dense and stable oxide layer (Ti dioxide, TiO2) with a thickness of 1.5-10 nm [13]. This TiO2 layer is responsible for the excellent biocompatibility of Ti implants because of its low level of electronic conductivity, high corrosion resistance, and thermodynamically stable state at physiological pH values [14-16]. However, the natural oxide layer can be easily broken and is not bioactive enough to form a direct bone-to-bone contact. Some studies have indicated that an artificial increase in the thickness of the native oxide layer will result in very strong reinforcement of the bone response [17]. Sundgren and I Lundstrom [18] assessed the implants retrieved from patients and demonstrated that successfully osseointegrated implants showed an increase in oxide thickness of up to 200 nm. Furthermore, Larsson et al. [6,7] found that there were no significant differences in the bone contact and bone formation between machined Ti implants (3-5 nm oxide thickness) and anodized implants (up to 200 nm oxide thickness).
Schreckenbach et al. [19] demonstrated some advantages of surface modification by anodic oxidation, such as the ability of fabricating porous Ti oxide films by dielectric breakdown, the changeability of the crystalline structure, and the chemical composition of the oxide film, depending on the fabrication conditions. Anodization can form a rough and homogeneous TiO2 layer with numerous nano-scale pores on the Ti surface, providing improved corrosion resistance and decreased ion release [20,21]. In addition, the pores on the layer provide an optimum biological environment for bone tissue through the ingrowth of mineralized tissue into the pore space and consequently improve osseointegration [22-24]. Furthermore, Bae et al. [25] reported that the nanotubular structure of pores can provide storage room for drugs, such as antibiotics, anti-inflammatory drugs, and growth factors, which might improve sustained drug delivery.
In summary, the anodic oxide film formed on the Ti surface is thought to improve the response of osteoblasts for osseointegration between the Ti surface and surrounding bone. However, the mechanical properties of the anodic oxide film formed on the surface of the Ti and the associated biological response of osteoblasts have not been completely understood. Thus, the purpose of this study was to evaluate the surface properties and cellular events occurring on an anodized Ti surface.
All specimens were kindly provided by the School of Materials Science and Engineering, Chonnam National University. Briefly, all commercially pure Ti (grade II, cp-Ti) samples were prepared as disks 15 or 25 mm in diameter and 1 mm in thickness. The cp-Ti disks were ground with 240 grit silicon carbide papers. These disks were ultrasonically degreased in acetone and ethanol for 10 minutes with deionized water rinsing between applications of each solvent. In the preliminary experiment in which the oxide film formed by anodic oxidation was evaluated for various durations (30-120 seconds), the specimens with 60 seconds of treatment showed excellent physical properties and were used as the test group in this study. The samples were then divided into 2 groups. Group I was a nontreated machined Ti surface. Group II was an anodized TiO2 surface using a constant voltage of 270 V for 60 seconds. The disks were anodized using pulse power (650 Hz). The electrolyte solution contained 0.15 M calcium acetate and 0.02 M calcium glycerophosphate.
The surface morphology of anodized Ti disks and their cross-sections were also observed by scanning electron microscopy (SEM; S-4700, Hitachi, Tokyo, Japan). Each group was examined with atomic force microscopy (AFM; Nano Scope-IIIa, Digital Instrument, Santa Barbara, CA, USA) to provide surface morphology information on the test surfaces. The surfaces of the anodized Ti disks were examined with an X-ray diffractometer (XRD; DMAX/1200, Rigaku, Tokyo, Japan). The contact angle of each sample was measured using an image analyzing microscope (Camscope, Sometech Inc., Seoul, Korea). The surface roughness was measured using a profilometer (Diavite DH-1, Asmeto AG, Richterswil, Switzerland). The measured surface roughness was represented by the roughness average, and five samples per group were used.
The samples for the corrosion test were embedded in a room temperature curing epoxy resin leaving an exposure area of 10 mm2×1 mm2. The control and test groups were exposed to the electrolyte. The electrolyte used was phosphate-buffered saline (PBS) at room temperature. A three-electrode cell set-up was used with a saturated calomel electrode, a platinum wire as reference, and a counter electrode.
A potentiodynamic polarization scan using a frequency response analyzer (EIS 300, Gamry Instruments Inc., Warminster, PA, USA) coupled to a potentiostat PCI4/300 (Gamry Instruments, Inc.), was acquired following 7 days of immersion in PBS.
All disks were placed under aseptic conditions in the bottom of 12- or 6-well culture dishes, then rinsed 3 times in 70% ethanol, exposed to ultraviolet light for 1 hour and air dried in a laminar flow.
Mouse MC3T3-E1 cells (ATCC, Rockville, MD, USA) were cultured in T-75 flasks in alpha minimum essential medium (α-MEM, Invitrogen, Carlsbad, CA, USA) supplemented with 10% heat-inactivated fetal bovine serum, 100 mg/mL penicillin, and 100 mg/mL streptomycin (Invitrogen) at 37℃ in a humidified atmosphere of 5% CO2-95% air.
Mouse MC3T3-E1 cells were evaluated for cell attachment and growth using SEM. Cells were seeded in a 12-well plate at a density of 1×104 cells/mL in α-MEM medium containing 10% FBS. After incubation for 3 days, the dishes were washed three times with PBS and fixed with 2.5% glutaraldehyde in 100 mM cacodylate buffer. The samples were dehydrated in increasing concentrations of ethanol (30%, 60%, 95%, and 100%), immersed in hexamethyldisilazane (Sigma-Aldrich Co., St. Louis, MO, USA) for 15 minutes, air-dried, and immediately mounted on aluminum stubs and coated with platinum.
Cells were cultured on machined Ti and three anodized TiO2 surfaces in 12-well plates at a density of 1×104 cells/mL in α-MEM. The control was cultured on a tissue culture plate. After 1 day, the medium was changed and the cells were cultured for an additional 2 days. Following incubation, the cell viability was assessed by 3-(4,5-dimethylthiazol-2-yl)-2,5-diphenyltetrazolium bromide (MTT) assay (CellTiter 96 Aqueous One, Promega Co., Madison, WI, USA). In these experiments, the formazan reduction was measured, which provides a useful tool for the measurement of individual cell viability and is directly proportional to the number of viable cells. The formazan accumulation was quantified by absorbance at 490 nm by an enzyme-linked immunosorbant assay plate reader (Microplate Manager, Bio-Rad Laboratories Inc., Hercules, CA, USA) and analyzed. All of the experiments were carried out in triplicate.
The assay for alkaline phosphatase (ALP) activity was carried out, to measure the ALP activity, the fetal rat calvarial cells were seeded on machined Ti and three anodized TiO2 surfaces in 12-well plates at a density of 1×104 cells/mL in BGJb media containing 10% FBS. Determination of ALP activity was performed at day 7. Briefly, cells were lysed in Triton 0.1% (Triton X-100) in PBS, then frozen at -20℃ and thawed. One hundred microliters of cell lysates was mixed with 200 mL of 10 mM p-nitrophenol phosphate and 100 mL of 1.5 M 2-amino-2-methyl-1-propanol buffer, and then incubated for 30 minutes at 37℃. The ALP activity was measured by an absorbance reading at 405 nm with a spectrophotometer (SmartSpec, Bio-Rad Laboratories Inc.). All of the experiments were carried out in triplicate.
One-way analysis of variance and Tukey test for repeated measurements were performed to examine the data for surface roughness, surface energy, contact angle measurement, cell proliferation, and ALP activity with the SPSS ver. 12.0 (SPSS Inc., Chicago, IL, USA). The results were considered significant where P<0.001.
Fig. 1 shows the surface morphology of machined Ti and anodized Ti. The anodized TiO2 surface showed relatively well-developed columnar structures and a porous oxide layer compared with the machined Ti surface. The cross-sectioned images showed that the TiO2 layer of the anodized Ti was thicker than the machined Ti (control, 17 nm; test, 1,500 nm) (Fig. 2). Fig. 3 shows the AFM images of the surface roughness, and the measurements revealed a root-mean-square of 0.24±0.05 µm and 0.28±0.04 µm, respectively (Table 1). The anodized TiO2 surface was found to be relatively rougher than the machined Ti surface, but there was no significance.
Fig. 4 shows an XRD pattern of the test group sample. The 60-second specimens showed many peaks indicative of anatase and rutile structures. This indicated that the Ti was further oxidized in the anodized TiO2 surface and formed oxide layers with rutile and anatase structures.
The contact angles are shown in Fig. 5. The water contact angle of the anodized TiO2 surface was significantly lower than the machined Ti surface (control, 87.20±3.01; test, 65.74±1.12) (P<0.001).
In order to evaluate the corrosion protection by the anodized TiO2 surface, a potentiodynamic polarization test was performed. Fig. 6 shows the curves for the two groups. From the polarization curves, the anodized TiO2 surface had superior corrosion resistance compared to the machined Ti surface. The curve of the anodized Ti surface showed nearly constant values of passive current density, indicating that its corrosion rate was in a steady state and that the passive film formed on its surfaces was stable.
The cell morphology was examined by SEM after 24 hours of cell seeding. Fig. 7 shows the SEM images of cell spreading. Under SEM, the cells adhered and grew well on the surfaces of the machined Ti surface and anodized TiO2 surface. The cells spread extensively and totally flattened on all of the anodized Ti surfaces. They were polygonal in shape and the individual cells were flat in appearance. On the machined Ti surface, the cells also spread polygonally and cell projections connecting cells were visible. There was no significant morphological difference between the two groups.
Fig. 8 shows the cell viability assessed by MTT assay. The number of cells gradually significantly increased as the cell incubation time increased in both groups (P<0.001). Although there was no significant difference in the cell viability between the two groups, the anodized Ti surface showed a tendency toward slightly higher viability than the machined Ti surface (P>0.001).
The assay for ALP activity is shown in Fig. 9. At 7 days, the ALP activity was significantly higher in the anodized Ti surface than in the machined Ti surface (P<0.001). The cells on the anodized Ti surface showed 47% higher ALP levels than those on the machined surface.
Since the anodization technique was introduced in the early 1930's, it has been widely studied as a method for enhancing the osseointegration between the implant surface and surrounding bone [3]. Some studies have shown that the improvement of bone contact and bone formation might be achieved by modification of the oxide thickness and surface topography [6,7]. Schreckenbach et al. [19] demonstrated some advantages of the anodic oxidation in surface modification, such as the ability to fabricate porous TiO2 films through dielectric breakdown, the changeability of the crystalline structure and chemical composition of the oxide film depending on the fabrication conditions providing improved corrosion resistance. Furthermore, Bae et al. [25] reported that the nanotubular structure of pores can provide storage room for drugs, such as antibiotics, anti-inflammatory drugs, and growth factors, which might improve sustained drug delivery. In the present study, we confirmed the advantages of an anodized Ti surface with regard to its surface characteristics and bioactivity. The surface properties were evaluated by SEM, XPS, and AFM and the cellular events on the anodized Ti surface were analyzed.
Various anodizing conditions have been tested to obtain the optimal surface modification of Ti in other studies. The anodizing conditions include the voltage, current, duration, and kind of electrolyte. Some studies have reported the results of anodizing used strong acids such as sulfuric acid and hydrofluoric acid [13]. These electrolytes are effective due to their electrolysis speed. However, they have disadvantages, such as harmful byproducts and difficult manipulation. Therefore, in this study, we used calcium acetate and glycerophosphate media, which has the properties of a weak acid. Furthermore, those electrolytes acted as a supplier of Ca and P, which the anodized Ti could contain in its oxide layer, which may benefit bone healing. Sixty seconds of anodization was used to form the oxide film because the specimens with 60 seconds of treatment showed good physical properties in our preliminary experiment. The formation of the oxide layer by anodization was verified by the XRD pattern.
Some aspects of surface characteristics can be modified by anodic oxidation of Ti. Anodization can increase the surface roughness of Ti and form micro pores and nanotube arrays of the porous crystalline oxide layer [11,26]. In this study, a rough, thick, and porous crystalline oxide layer formed by anodization was demonstrated by SEM imaging and AFM assay. The SEM images of the cross-sections of the samples showed that the thickness of the oxide layer was 1,500 nm, which was thought to be appropriate in the cell responses in our report. Several authors investigated the relationship of the thickness of the oxide film and the biocompatibility of the Ti implants. Lasson et al. [6,7] showed that a thicker surface oxide layer had an enhancing effect on the rate of bone formation and thick oxide (180 nm in thickness) showed a tendency toward a higher degree of bone contact with the implant and bone area within the threads. Furthermore, Sul et al. [24] demonstrated that electrochemically reinforced oxide surfaces with an oxide thickness of 600, 900, or 1,000 nm enhanced the bone anchorage of such implants in comparison with Ti implants with an oxide thickness of less than 200 nm in terms of the removal torque and resonance frequency analysis. In our study, compared to other studies, a thicker oxide layer was obtained, and this may have contributed to better physical properties. Yao and Webster [11] demonstrated that the pore size could be determined by the current densities, applied potential, and electrolyte concentrations, and varied from a few tens of nanometers to a few hundred nanometers. In their study, the size of the nano-tubes was about 70 nm and it promoted the calcium deposition by osteoblasts. In this study, the inner diameter of the pore structure was between about 90 nm and 200 nm, which was similar to the results of Yao and Webster' study.
In addition, corrosion resistance occurring in vivo plays an important role in the life of the implant [20,24]. In this study, anodizing increased the oxide thickness and consequently improved corrosion protection. In agreement with the present study, several studies have suggested that the corrosion resistance of implants could be improved by anodization because the thickness of the protective oxide layer increased, and this resulted in less metal ion release in the human body [22]. The improvement of corrosion resistance is important in view of its biocompatibility. The physiological solution (body fluid) is considered extremely corrosive to metallic material. The possible release of toxic metallic ions and/or particles through corrosion or wear processes leads to inflammatory cascades that can reduce biocompatibility and cause tissue loss. In the case of the Ti-6Al-4V alloy, the vanadium ions and aluminum ions are dissolved from the implant surface and are associated with serious health problems, adverse tissue reactions, and potential neurological disorders [20,24]. Therefore, it is important to provide an effective surface coating technology for Ti alloys. In this report, thick oxide film (1,500 nm) obtained by oxidization may block the emission of those harmful ions, prevent complications, and contribute to a good biologic response.
As the water contact angle is lower (i.e., high hydrophilic property), the wettability is increased, and this is generally favorable in biocompatibility [27,28]. Eriksson et al. [29] demonstrated that the increase in wettability promoted the interaction between the implant surface and the biological environment, and they reported that cell-surface interactions are influenced by surface energy. Furthermore, cell activation was more rapid on hydrophilic surfaces [30]. As shown in other studies, the present study showed that the anodized Ti surface had a lower contact angle than the machined Ti surface. This lower contact angle may create a hydroxylated and hydrophilic surface and promote the adhesion of relevant proteins.
The crystal structure of the oxide layer was analyzed by assessing the X-ray diffraction pattern. In this study, the anodized Ti surface had a stronger anatase peak at the same degree than the machined Ti surface. It is well known that TiO2 has three crystal structures: anatase, rutile, and brookite and anatase TiO2 is more reactive than rutile. Oh et al. [31] reported that as the anodic reaction increased, the X-ray peak intensities of anatase gradually increased, and the crystalline phase of anodic films was predominantly anatase.
Most recent studies of surface roughness have focused on cell attachment and osseointegration and have shown better results on rough surfaces [18,20]. However, the present study showed that the roughness was similar between the two groups (control, 0.24±0.05 µm; test, 0.28±0.04 µm) and this suggests that the surface roughness may not be what is influencing the cell response. The SEM images showed that the cells were plated well on the anodized Ti surface, and this was similar to those on the machined surfaces. We also evaluated the cell viability and proliferation level by MTT assay. The cells of all of the samples proliferated actively during the experimental period. Although there was no statistical difference between the groups, the anodized Ti surface showed a tendency toward higher proliferation of osteoblasts than the machined Ti surface. This result suggests that the roughness did not affect the cell proliferation, and the procedure for anodization showed an absence of cytotoxicity in cell attachment. A possible explanation of this result is the mechanical properties of the anodized Ti surface; it had a lower contact angle and stronger anatase peak than the machined Ti surface.
Furthermore, ALP activity was assessed to evaluate the cell response to the anodized Ti surface. ALP is well known as a biomarker specific to the initial differentiation of osteoblasts [28,29]. In this study, the cells on the anodized Ti surface showed significantly higher ALP activity levels than the cells in the control group. This indicated that the anodization procedure appeared to affect the ALP activity, and the improved ALP activity suggested the facilitation of differentiation of the fetal rat calvarial cells into osteoblasts. This result was similar to other reports in which an anodized surface showed higher ALP activity [25,32,33]. Bae et al. [25] showed that the ALP activity in an anodized group was 55% higher than that of the untreated group. Zhao et al. [32] noted that the reason for this result could be that the anodized Ti was more hydrophilic and contained many Ti-OH groups on its surface layer.
Although it was well conducted, the present study has limitations. We evaluated the biocompatibility of the anodized TiO2 surface with osteoblast-like cells, and the cell response was improved at the anodized TiO2 surface within 7 days of cell culture. However, this effect was not evaluated in vivo for a longer period. To confirm the improved osseointegration of anodized Ti, an in vivo study should be performed. In summary, the anodized TiO2 surface had a higher level of cell proliferation and ALP activity than a machined surface. These properties could promote the bone response around the implant and improve osseointegration. Consequently, they should contribute to the long-term success rate of dental implants.
Figures and Tables
Figure 1
Scanning electron microscopy. (A) The machined titanium (Ti) surface showed a uniform texture. (B) The anodized Ti surface showed relatively well-developed columnar structures and a porous oxide layer.
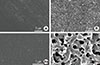
Figure 2
Scanning electron microscope images of the cross-sectioned titanium. (A) The machined titanium (Ti) surface. (B) The anodized Ti dioxide (TiO2) surface. The thickness of the TiO2 layer was thicker on the anodized titanium than the machined Ti.
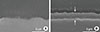
Figure 3
Atomic force microscopy (AFM) analysis of (A) the machined titanium (Ti) surface and (B) the anodized Ti dioxide (TiO2) surface. Three-dimensional AFM images (10 µm×10 µm) showed nanoscale roughness on the anodized TiO2 surface.
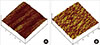
Figure 4
X-ray photoelectron spectroscopy analysis. (A) The machined titanium (Ti) surface. (B) The anodized Ti dioxide (TiO2) surface. The anodized TiO2 surface showed a stronger anatase peak at the same degree than the machined Ti surface.
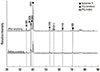
Figure 5
The contact angle of (A) the machined titanium (Ti) surface and (B) the anodized Ti dioxide (TiO2) surface examined by an image analyzing microscope. The water contact angle of the anodized TiO2 surface was significantly lower than that of the machined Ti surface. *A statistically significant difference as compared with machined Ti (P<0.001).
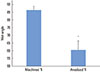
Figure 6
Corrosion resistance measured by potentiodynamic polarization curves of (A) the machined titanium (Ti) surface and (B) the anodized Ti dioxide surface. The curve of the anodized Ti surface showed nearly constant values of passive current density.
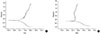
Figure 7
Cell adhesion examined by scanning electron microscopy. The cells adhered and grew well on the surfaces of (A) the machined titanium (Ti) surface and (B) the anodized Ti dioxide surface.
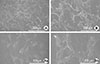
Figure 8
Cell viability measured by the 3-(4,5-dimethylthiazol-2-yl)-2,5-diphenyltetrazolium bromide assay of (A) the machined titanium (Ti) surface and (B) the anodized Ti dioxide surface. The number of cells gradually significantly increased as the cell incubation time increased in both groups. The anodized Ti surface showed a tendency toward higher cell proliferation than the machined Ti surface.
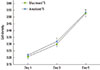
Figure 9
The alkaline phosphatase activity of the machined titanium (Ti) and anodized Ti dioxide (TiO2) surface. The ALP activity in the anodized TiO2 surface was significantly higher than that of the machined Ti surface. *A statistically significant difference as compared with machined Ti (P<0.001).
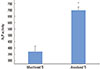
ACKNOWLEDGEMENTS
This study was supported by grant (CRI 11006-1) of the Chonnam National University Hospital Research Institute of Clinical Medicine.
References
1. Uzumaki ET, Santos AR, Lambert CS. Titanium oxide (TiO2) coatings produced on titanium by oxygen plasma immersion and cell behavior on TiO2. Key Eng Mater. 2006; 18:367–370.
2. Kasemo B. Biocompatibility of titanium implants: surface science aspects. J Prosthet Dent. 1983; 49:832–837.


3. Baier RE, Meenaghan MA, Hartman LC, Wirth JE, Flynn HE, Meyer AE, et al. Implant surface characteristics and tissue interaction. J Oral Implantol. 1988; 13:594–606.
4. Ong JL, Prince CW, Raikar GN, Lucas LC. Effect of surface topography of titanium on surface chemistry and cellular response. Implant Dent. 1996; 5:83–88.


5. Placko HE, Mishra S, Weimer JJ, Lucas LC. Surface characterization of titanium-based implant materials. Int J Oral Maxillofac Implants. 2000; 15:355–363.
6. Larsson C, Thomsen P, Lausmaa J, Rodahl M, Kasemo B, Ericson LE. Bone response to surface modified titanium implants: studies on electropolished implants with different oxide thicknesses and morphology. Biomaterials. 1994; 15:1062–1074.


7. Larsson C, Thomsen P, Aronsson BO, Rodahl M, Lausmaa J, Kasemo B, et al. Bone response to surface-modified titanium implants: studies on the early tissue response to machined and electropolished implants with different oxide thicknesses. Biomaterials. 1996; 17:605–616.


8. Lausmaa J. Mechanical, thermal, chemical and electrochemical surface treatment of titanium. In : Brunette DM, Tengvall P, Textor M, Thomsen P, editors. Titanium in medicine. New York: Springer;2011. p. 231–266.
9. Sittig C, Textor M, Spencer ND, Wieland M, Vallotton PH. Surface characterization of implant materials c.p. Ti, Ti-6Al-7Nb and Ti-6Al-4V with different pretreatments. J Mater Sci Mater Med. 1999; 10:35–46.
10. Bordji K, Jouzeau JY, Mainard D, Payan E, Netter P, Rie KT, et al. Cytocompatibility of Ti-6Al-4V and Ti-5Al-2.5Fe alloys according to three surface treatments, using human fibroblasts and osteoblasts. Biomaterials. 1996; 17:929–940.


11. Yao C, Webster TJ. Anodization: a promising nano-modification technique of titanium implants for orthopedic applications. J Nanosci Nanotechnol. 2006; 6:2682–2692.


12. Sato M, Webster TJ. Nanobiotechnology: implications for the future of nanotechnology in orthopedic applications. Expert Rev Med Devices. 2004; 1:105–114.


13. Sul YT, Johansson CB, Jeong Y, Roser K, Wennerberg A, Albrektsson T. Oxidized implants and their influence on the bone response. J Mater Sci Mater Med. 2001; 12:1025–1031.
14. Sul YT, Byon ES, Jeong Y. Biomechanical measurements of calcium-incorporated oxidized implants in rabbit bone: effect of calcium surface chemistry of a novel implant. Clin Implant Dent Relat Res. 2004; 6:101–110.


15. Sul YT, Johansson C, Wennerberg A, Cho LR, Chang BS, Albrektsson T. Optimum surface properties of oxidized implants for reinforcement of osseointegration: surface chemistry, oxide thickness, porosity, roughness, and crystal structure. Int J Oral Maxillofac Implants. 2005; 20:349–359.
16. Sul YT, Johansson C, Byon E, Albrektsson T. The bone response of oxidized bioactive and non-bioactive titanium implants. Biomaterials. 2005; 26:6720–6730.


17. Sunny MC, Sharma CP. Titanium-protein interaction: changes with oxide layer thickness. J Biomater Appl. 1991; 6:89–98.


18. Sundgren JE, I Lundstrom BP. Auger electron spectroscopic studies of the interface between human tissue and implants of titanium and stainless steel. J Colloid Interface Sci. 1986; 110:9–20.


19. Schreckenbach JP, Marx G, Schlottig F, Textor M, Spencer ND. Characterization of anodic spark-converted titanium surfaces for biomedical applications. J Mater Sci Mater Med. 1999; 10:453–457.
20. Jeong YH, Choe HC, Brantley WA. Corrosion characteristics of anodized Ti-(10-40wt%)Hf alloys for metallic biomaterials use. J Mater Sci Mater Med. 2011; 22:41–50.


21. Song HJ, Kim MK, Jung GC, Vang MS, Park YJ. The effects of spark anodizing treatment of pure titanium metals and titanium alloys on corrosion characteristics. Surf Coat Technol. 2007; 201:8738–8745.


22. Yao C, Slamovich EB, Webster TJ. Enhanced osteoblast functions on anodized titanium with nanotube-like structures. J Biomed Mater Res A. 2008; 85:157–166.


23. Sul YT. The significance of the surface properties of oxidized titanium to the bone response: special emphasis on potential biochemical bonding of oxidized titanium implant. Biomaterials. 2003; 24:3893–3907.


24. Sul YT, Johansson CB, Jeong Y, Wennerberg A, Albrektsson T. Resonance frequency and removal torque analysis of implants with turned and anodized surface oxides. Clin Oral Implants Res. 2002; 13:252–259.


25. Bae IH, Yun KD, Kim HS, Jeong BC, Lim HP, Park SW, et al. Anodic oxidized nanotubular titanium implants enhance bone morphogenetic protein-2 delivery. J Biomed Mater Res B Appl Biomater. 2010; 93:484–491.


26. Balasundaram G, Yao C, Webster TJ. TiO2 nanotubes functionalized with regions of bone morphogenetic protein-2 increases osteoblast adhesion. J Biomed Mater Res A. 2008; 84:447–453.
27. Das K, Bose S, Bandyopadhyay A. Surface modifications and cell-materials interactions with anodized Ti. Acta Biomater. 2007; 3:573–585.


28. Webb K, Hlady V, Tresco PA. Relative importance of surface wettability and charged functional groups on NIH 3T3 fibroblast attachment, spreading, and cytoskeletal organization. J Biomed Mater Res. 1998; 41:422–430.


29. Eriksson C, Nygren H, Ohlson K. Implantation of hydrophilic and hydrophobic titanium discs in rat tibia: cellular reactions on the surfaces during the first 3 weeks in bone. Biomaterials. 2004; 25:4759–4766.


30. Eriksson C, Nygren H. Polymorphonuclear leukocytes in coagulating whole blood recognize hydrophilic and hydrophobic titanium surfaces by different adhesion receptors and show different patterns of receptor expression. J Lab Clin Med. 2001; 137:296–302.


31. Oh HJ, Lee JH, Jeong Y, Kim YJ, Chi CS. Microstructural characterization of biomedical titanium oxide film fabricated by electrochemical method. Surf Coat Technol. 2005; 198:247–252.

