Abstract
Objective
To determine the unique contribution of geometrical design characteristics of orthodontic mini-implants on maximum insertion torque while controlling for the influence of cortical bone thickness.
Methods
Total number of 100 cylindrical orthodontic mini-implants was used. Geometrical design characteristics of ten specimens of ten types of cylindrical self-drilling orthodontic mini-implants (Ortho Easy®, Aarhus, and Dual Top™) with diameters ranging from 1.4 to 2.0 mm and lengths of 6 and 8 mm were measured. Maximum insertion torque was recorded during manual insertion of mini-implants into bone samples. Cortical bone thickness was measured. Retrieved data were analyzed in a multiple regression model.
Versatile temporary anchorage device (TAD) systems offer fixed anchorage to improve the efficiency of orthodontic treatment. Orthodontic mini-implants are proven to be very helpful as temporary skeletal anchorage, particularly in patients with low compliance or insufficient dental structures.1,2 Miniscrew-type implants are useful because they are easy to insert and to remove in a wide range of areas, their surgical implantation is less traumatic, they can be used for various purposes, immediate orthodontic loading is possible, and their associated cost is low.3,4
Successful orthodontic treatment greatly depends on appropriate, stable anchorage. It was reported that mini-implants have a lower success rate (80-85%) than osseointegrated implants (91.00% and 97.81%, for maxilla and mandible, respectively).5 The displacement of a screw can occur due to inflammation of surrounding tissue or as a result of a poor bone-screw interface. Suggested factors that cause degeneration of the bone at the implant-tissue interface include excessive insertion torque, heat at the border between the screw and bone, and mechanical injury.6 Therefore, the recommended optimal maximum insertion torque (MIT) values between 50-100 Nmm help clinicians improve clinical results.7 The initial implant stability is important for clinical success if TADs are to be loaded immediately.6 In cases where primary stability is not achieved during insertion, the mini-implant cannot be engaged in therapy for the next 6-8 weeks, which prolongs orthodontic treatment.
Many studies have been conducted to analyze MIT with respect to the diameter and length of the miniscrew.8,9,10 Manufacturers offer their TADs mainly with handheld screwdrivers (Figure 1), which widens their applicability. The intentions of this study are to obtain data in conditions similar to those of routine in-office procedures and to determine critical factors of the screw design that could be controlled to avoid failure of TADs in a manual insertion procedure. Our goal is to determine the unique contribution of individual geometrical design characteristics of orthodontic mini-implants-thread length and outer diameter, depth/pitch ratio (thread shape factor [TSF]), and lead angle of thread-on MIT while controlling for the influence of cortical bone thickness.
We hypothesize that diameter, TSF, and lead angle of the thread are significant predictors of MIT.
The following ten cylindrical self-drilling orthodontic mini-implant types with a bracket-like head (all made of titanium alloy grade 5, Ti-6Al-4V) were investigated in this study (Figure 2): Ortho Easy® (FORESTADENT®, Pforzheim, Germany), 1.7 × 6 mm and 1.7 × 8 mm; Aarhus Anchorage System (MEDICON eG, Tuttlingen, Germany), 1.5 × 6 mm and 1.5 × 8 mm; and Jeil Dual Top™ Anchor System (Jeil Medical Corp., Seoul, Korea), 1.4 × 6 mm, 1.6 × 6 mm, 2.0 × 6 mm, 1.4 × 8 mm, 1.6 × 8 mm, and 2.0 × 8 mm.
Ten specimens of each mini-implant type were tested. Precise measurement of all orthodontic miniscrews was conducted using the Olympus SZX16® optical stereomicroscope (Olympus Corporation, Tokyo, Japan) with 8× and 20× magnifications. Each microscopic sample was photographed alongside a calibrated chart, and the measurement was read on photographs. Length, outer diameter, depth, pitch, and lead angle of the screw thread were the geometrical design characteristics measured in this study (Figure 3). All implants were found to have an asymmetric overall thread shape type. TSF was calculated as the ratio of thread depth to pitch for each miniscrew.
One hundred orthodontic mini-implants were manually inserted perpendicular to the bone surface using the handheld screwdriver of the respective mini-implant system without pre-drilling of a pilot hole. Each miniimplant was screwed into one bone sample. The insertion torque was measured for the duration of the insertion up to the implant collar, and measured values of MIT (expressed as Nmm) were digitalized and saved using PicoLog® 5.14.6 (Pico® Technology Ltd., St. Neots, UK) data acquisition software. The torque-measuring device (Institut IGH, Zagreb, Croatia) consisted of a fixed body with four sensors connected to a high resolution PicoScope® oscilloscope (Pico® Technology Ltd.) and a custom made docking unit for the bone samples (Figure 4).
Each bone sample measuring 2 × 1.5 cm in size was prepared from the spinal aspect of swine ribs. Fresh swine ribs were deperiosted, cut into bone samples with a water-cooling surgical engine, and stored in physiological solution at 4℃ until further evaluation. Cortical bone thickness was measured at both cross-sectioned ends of each bone sample using a digital sliding caliper (Levior s.r.o., Prerov, Czech Republic) with an accuracy of ± 0.03 mm. The mean value of the cortical bone thickness measurements of each sample was used for further statistical analysis.
Institutional review board approval was not needed for this study.
Using MIT as the outcome, Pearson's bivariate correlation coefficient was used to measure the strength of the association between screw design parameters and cortical thickness when not controlling for the influence of other variables. A multiple linear regression analysis was used to determine the unique contribution of individual geometrical design characteristics of orthodontic mini-implants in predicting the MIT while controlling for other design variables and cortical bone thickness. The presence of multicollinearity was evaluated with the help of a variance inflation factor (VIF) and tolerance. Statistical analyses were carried out using SPSS® version 10.0 statistics software (SPSS Inc., Chicago, IL, USA). Statistical significance was preset to p < 0.05.
Geometrical design characteristics of tested orthodontic miniscrews assessed using the optical stereomicroscope are shown in Table 1. Cortical thickness of bone samples ranged from 0.7-3.1 mm (mean 1.5 ± 0.4 mm). The MIT values were between 50.86-347.49 Nmm (122.01 ± 49.03 Nmm).
Descriptive statistics for MIT with length and outer diameter of the screw thread evaluated at covariate cortical bone thickness (1.5190 mm) are shown in Table 2.
In bivariate correlations, MIT was significantly positively correlated with the depth of the thread (r = 0.413; p < 0.001), the pitch of the thread (r = 0.402; p < 0.001), TSF (r = 0.403; p < 0.001), the outer diameter of the implant thread (r = 0.398; p < 0.001), and cortical bone thickness (r = 0.409; p < 0.001), whereas it was not significantly correlated with the length of the implant thread or the lead angle of the thread.
The multiple linear regression model showed that while controlling for other variables, significant predictors for higher MIT were a larger implant diameter (p < 0.001), a higher lead angle (p < 0.001), and thicker cortical bone (p < 0.001; Table 3). The unique contribution of these factors in accounting for the MIT variability was 12.3, 10.7, and 24.7%, respectively. The whole model accounted for 44.2% of the variability in MIT.
In-office decision making regarding selection of a specific implant type is related to host characteristics, namely, bone density and cortical thickness,11 and choosing the most appropriate geometrical screw design combination will enhance the primary stability of the TAD and thus, the success of orthodontic therapy.
Our study showed high variability in the MIT in the manual procedure of miniscrew insertion but revealed that significant unique contributors to higher MIT values were thicker cortical bone, a larger outer implant thread diameter, and a higher thread lead angle.
Similar conclusions regarding cortical bone thickness and implant diameter as important predictors for MIT are found in other studies.8,9,10,12 It has been noted that overall implant shape (conical vs. cylindrical) influences MIT,12 but the unique predictive value of specific geometrical design characteristics has not been analyzed in detail previously.
In bivariate correlations, statistically significant correlations were found between MIT and the depth of the thread, the pitch of the thread and TSF, but not between MIT and lead angle of the thread.
These results indicated an association between two variables without controlling for the influence of other possible cofactors. Therefore, a multiple regression model was included to control for the influence of other possible cofactors. This advanced model established no significant predictive value for TSF but strengthened the predictive value of cortical bone thickness and confirmed a positive correlation with lead angle, whose unique contribution in the prediction of MIT was 10.7%. This multiple regression model explains only 44.2% of the MIT prediction, leaving a large portion of the variability of MIT unaccounted for. We used the forced-entry regression method, where all predictors are forced into the model simultaneously. This method is appropriate for theory testing13 because the experimenter does not determine the entrance order of variables. Predictors are chosen based on previous studies and analysis of intercorrelations and multicollinearity.9,10,12,14,15 Further studies might include bone density, angle of implantation, and changes in implant design when trying to combine the best individual features to maximize implant efficiency.
During the statistical analysis, we encountered the problem of multicollinearity because the lead angle correlated with the outer diameter and pitch of the thread, TSF correlated to depth and pitch of the thread, and the depth of the thread correlated with the outer diameter. Therefore, depth and pitch of the thread were not accounted for in the multiple regression analysis; instead, TSF was taken into account as a combined value of both variables. The depth/pitch ratio (TSF) is not linearly related to changes in lead angle. It seems that higher lead angle improves cutting efficiency during implantation because the lead defines the axial travel for a single revolution,15 whereas a larger thread surface (represented through TSF) is more resistant to pullout forces.8,9
Values for the length and outer diameter of the screw thread are provided by every manufacturer, but the manufacturer's data regarding depth, pitch, and lead angle of the thread are rarely provided. Results of all measured geometrical design characteristics show very small variations within the same screw type, making their use highly recommended. It should be noted that the actual size of the outer thread diameter of the tested Aarhus Anchorage System mini-implants (1.5 × 6 mm; 1.5 × 8 mm) is close to the diameter of the tested Dual Top™ mini-implants (1.6 × 6 mm; 1.6 × 8 mm); thus, using mini-implants of different sizes may not be so different in actual in-office work. The greater value of the larger implant should be taken into account when implanting near tooth roots.
All implantations were done manually with no pilot hole because all of the manufacturers recommend direct implantation for self-drilling mini-implants in most bony areas in the oral cavity, except for mandibular and palatal areas, where use of a pilot drill or cortical bone punch is recommended because of the presence of dense cortical bone. Many researchers recommend insertion without a guide hole for self-drilling implants with diameters up to 1.6 mm.7 Others state that a diameter smaller than 1.5 mm significantly increases the risk for implant fracture.16 Several studies showed that self-drilling mini-implants have better primary stability when inserted without a pilot hole.2,14 The majority of routine in-office orthodontic mini-implants are placed manually. Additionally, the measurement of MIT is not possible in every situation, and orthodontic implantation often proceeds until no thread is visible and the transmucosal neck is seated in the surrounding soft tissue. Therefore, practitioners have only one "measure" for primary stability available, which is feeling the resistance, especially the final resistance, of the bone during insertion, which corresponds to the MIT. Results for the MIT in this study are somewhat higher compared to previously recommended insertion torques7 but are far below the fracture torque values of the respective mini-implant types shown in another study.17 These values are in agreement with the recommendations for using a pilot hole for larger diameters in order to achieve better long-term stability (secondary stability) because excessive insertion forces could cause later bone necrosis.9 The representative curve for manual insertion of orthodontic mini-implants shows oscillations in insertion torque as a result of the repositioning of the screwdriver in the surgeon's hand (Figure 5). Intermittent force,18 lower speed,19 and smaller cortical thickness20 reduce the chance for heat production at the border between the screw and bone, which is a known factor for the failure of implantation. On the other hand, during manual implantation, excessive insertion torque and mechanical injury can be controlled only by the operator's subjective judgment. Although the motor-driven method of insertion showed better results, it is not recommended for beginners and is more complicated and expensive overall.21 Therefore, one should control MIT by combining data from the implantation site (available space, cortical bone thickness) and implant design characteristics.
It is widely accepted that MIT is an important and measurable factor of primary stability of orthodontic miniscrews6,14 because other measurements described in the literature-radiological examination, Periotest® (Medizintechnik Gulden, Modautal, Germany), and removal torque8-deliver insufficiently precise measurements or have limited areas of application,22 while the resonance frequency analysis (RFA) by Osstell™ (Integration Diagnostics Ltd., Goteborgsvagen, Sweden) is currently not applicable for orthodontic mini-implants.23
This study and others23,24,25,26 used swine ribs because their ratio between cortical and spongious bone is similar to those in the alveolar processes of the maxilla and mandible. The cortical bone thickness was on average 1.5 ± 0.4 mm, which represents variations in tested biological material similar to the range in human jaw bones and enables analysis of the influence of variability of cortical bone thickness on overall MIT values.11,25 The primary stability of a miniscrew is important for immediate loading, and because it is intended for temporary use, it is desirable to achieve good primary stability.6,7,9 Findings from this study indicate possible choices regarding implant design and host characteristics in order to achieve proper primary stability without compromising long-term stability because all measurements were conducted in simulated in-office conditions. Our analysis of various implant types showed that lead angle may be an important factor of MIT values, so manufacturers should consider increasing the lead angle of the implant to improve the implant design and achieve better primary stability in cases where the operator cannot use a larger implant diameter.
Figures and Tables
Figure 2
Tested mini-implants (from left to right): Dual Top™ (Jeil Medical Corp., Seoul, Korea) 1.4 × 6 mm, and 1.4 × 8 mm; Aarhus (MEDICON eG, Tuttlingen, Germany) 1.5 × 6 mm, and 1.5 × 8 mm; Dual Top™ 1.6 × 6 mm, and 1.6 × 8 mm; Ortho Easy® (FORESTADENT®, Pforzheim, Germany) 1.7 × 6 mm, and 1.7 × 8 mm; Dual Top™ 2.0 × 6 mm, and 2.0 × 8 mm.

Figure 3
Geometrical design characteristics of mini-implants (length [a], outer diameter [b], depth [c], pitch [d], and lead angle [e] of the thread).
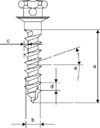
Table 1
Geometrical design characteristics of orthodontic mini-implants assessed with optical stereomicroscope
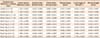
ACKNOWLEDGMENTS
A part of tested mini-implants was donated by the manufacturers FORESTADENT, MEDICON eG, and Jeil Medical Corp., to whom authors hereby thank for the donation.
This research is part of the University of Rijeka research project 13.06.2.1.53, principal investigator Stjepan Špalj.
References
1. Kanomi R. Mini-implant for orthodontic anchorage. J Clin Orthod. 1997; 31:763–767.
3. Tseng YC, Hsieh CH, Chen CH, Shen YS, Huang IY, Chen CM. The application of mini-implants for orthodontic anchorage. Int J Oral Maxillofac Surg. 2006; 35:704–707.


4. Poggio PM, Incorvati C, Velo S, Carano A. "Safe zones": a guide for miniscrew positioning in the maxillary and mandibular arch. Angle Orthod. 2006; 76:191–197.
5. Cheng SJ, Tseng IY, Lee JJ, Kok SH. A prospective study of the risk factors associated with failure of mini-implants used for orthodontic anchorage. Int J Oral Maxillofac Implants. 2004; 19:100–106.
6. Meredith N. Assessment of implant stability as a prognostic determinant. Int J Prosthodont. 1998; 11:491–501.
7. Motoyoshi M, Hirabayashi M, Uemura M, Shimizu N. Recommended placement torque when tightening an orthodontic mini-implant. Clin Oral Implants Res. 2006; 17:109–114.


8. Pithon MM, Nojima MG, Nojima LI. In vitro evaluation of insertion and removal torques of orthodontic mini-implants. Int J Oral Maxillofac Surg. 2011; 40:80–85.


9. Wilmes B, Drescher D. Impact of bone quality, implant type, and implantation site preparation on insertion torques of mini-implants used for orthodontic anchorage. Int J Oral Maxillofac Surg. 2011; 40:697–703.


10. Lim SA, Cha JY, Hwang CJ. Insertion torque of orthodontic miniscrews according to changes in shape, diameter and length. Angle Orthod. 2008; 78:234–240.


11. Xu Z, Zhao L, Wu Y, Wei X, Wang J, Tang N, et al. Histomorphometric and biomechanical analyses of the osseointegration of loaded orthodontic microscrews inserted at different cortical bone thickness sites. Oral Surg Oral Med Oral Pathol Oral Radiol. 2012; doi: 10.1016/j.oooo.2012.06.007[Epub ahead of print].


12. Pithon MM, Nojima MG, Nojima LI. Primary stability of orthodontic mini-implants inserted into maxilla and mandible of swine. Oral Surg Oral Med Oral Pathol Oral Radiol. 2012; 113:748–754.


13. Studenmund AH, Cassidy HJ. Instructor's manual to accompany. Using econometrics, a practical guide. Boston: Little Brown;1987.
14. Miyawaki S, Koyama I, Inoue M, Mishima K, Sugahara T, Takano-Yamamoto T. Factors associated with the stability of titanium screws placed in the posterior region for orthodontic anchorage. Am J Orthod Dentofacial Orthop. 2003; 124:373–378.


15. American Gear Manufacturers Association, American National Standards Institute. Gear nomenclature, definitions of terms with symbols: AGMA standard. Alexandria: American Gear Manufacturers Association;2005.
16. Dalstra M, Cattaneo PM, Melsen B. Load transfer of miniscrews for orthodontic anchorage. Orthodontics. 2004; 1:53–62.
17. Wilmes B, Panayotidis A, Drescher D. Fracture resistance of orthodontic mini-implants: a biomechanical in vitro study. Eur J Orthod. 2011; 33:396–401.


18. Kondo S, Okada Y, Iseki H, Hori T, Takakura K, Kobayashi A, et al. Thermological study of drilling bone tissue with a high-speed drill. Neurosurgery. 2000; 46:1162–1168.


19. Brisman DL. The effect of speed, pressure, and time on bone temperature during the drilling of implant sites. Int J Oral Maxillofac Implants. 1996; 11:35–37.
20. Toews AR, Bailey JV, Townsend HG, Barber SM. Effect of feed rate and drill speed on temperatures in equine cortical bone. Am J Vet Res. 1999; 60:942–944.
21. Kim JS, Choi SH, Cha SK, Kim JH, Lee HJ, Yeom SS, et al. Comparison of success rates of orthodontic mini-screws by the insertion method. Korean J Orthod. 2012; 42:242–248.


22. Cha JY, Yu HS, Hwang CJ. The validation of Periotest values for the evaluation of orthodontic mini-implants' stability. Korean J Orthod. 2010; 40:167–175.


23. Aparicio C, Lang NP, Rangert B. Validity and clinical significance of biomechanical testing of implant/bone interface. Clin Oral Implants Res. 2006; 17:Suppl 2. 2–7.


24. Kim SJ, Yoo J, Kim YS, Shin SW. Temperature change in pig rib bone during implant site preparation by low-speed drilling. J Appl Oral Sci. 2010; 18:522–527.

