Abstract
Objective
The aims of this study were to examine whether a passive stretch stimulus by means of a functional appliance induces changes in the fiber composition of masticatory muscles and whether these changes are similar to the changes in stretched limb muscle fibers by using RT-PCR, western blot, and immunohistochemical assays.
Methods
Five male New Zealand White rabbits were fitted with a prefabricated inclined plane on the maxillary central incisors to force the mandible forward (- 2 mm) and downward (- 4 mm). Further, 1 hind limb was extended and constrained with a cast so that the extensor digitorum longus (EDL) was stretched when the animal used the limb. The animals were sacrificed after 1 week and the masseter, lateral pterygoid, and EDL were processed and compared with those from control animals (n = 3).
Microstructural differences between the jaw-closing and the limb muscles are well studied. When a limb muscle is stretched, changes in the expression of myosin heavy chain (MyHC), the protein primarily responsible for the contractile speed and metabolism type of muscle fibers, are observable within days.1 When stretched, muscle fibers tend to change from a fast phenotype (mainly comprising MyHC IIb) to a slow phenotype (consisting mainly of MyHC Iβ or rarely Iα).1-6 These changes, although controversial, occur along a continuum of changes in the expression of MyHCs from isoform IIb to iso form I.7 In addition, the expression of less common isoforms, such as embryonic or neonatal MyHCs (developmental MyHCs), may also be increased.8
The same responses might occur in the masticatory muscles after an increase in occlusal vertical dimension and/or mandibular advancement.9,10 In animal models, functional appliances commonly used for orthodontic treatment induce a phenotypic change in masticatory muscle fibers, with a tendency toward transition to a slow phenotype.11,12 Slow fibers are fatigue resistant and easily convertible to fast fibers; in comparison, fast fibers are fatigable but difficult to convert to slow fibers. Therefore, in a masticatory muscle stretched by a functional appliance, the fiber composition would gradually balance as the orofacial structures grow and then, treatment effects would be sustained without relapse provided that the new fiber proportion is maintained. However, a phenotypic change appears to occur only within the fast fiber population, and a definite molecular change among slow fibers remains controversial.9,10,13
The aims of this study were to examine whether a passive stretch stimulus by means of a functional appliance induces changes in the fiber composition of masticatory muscles and whether these changes are similar to the changes in stretched leg muscle fibers by using RT-PCR, western blot, and immunohistochemical assays.
Eight male growing New Zealand White rabbits with an average weight of 2.5 kg and average age of 11 weeks were divided into control and experimental groups, consisting of 3 and 5 rabbits, respectively. Under the assumption that the MyHC composition in the masticatory muscles of the control animals would vary less than that in the experimental animals, the control sample size was minimized. The rabbits were brought to the institutional animal facility and allowed to acclimate for 2 weeks. In the experimental group, each animal was sedated with ketamine (40 mg/kg I.M.) and xylazine (5 mg/kg I.M.), the maxillary central incisors were etched with 37% phosphoric acid, and a prefabricated nickel-chromium inclined plane was fitted on these teeth (Figure 1) by using glass ionomer cement (Principle®; Dentsply Caulk, Milford, DE, USA). This inclined-plane appliance was designed to force the mandible approximately 2 mm forward and approximately 4 mm downward. The control group did not receive this appliance.11
While sedated, the experimental and control animals also received a cast (Vetcast®; 3M, St. Paul, MN, USA) to stretch 1 hind limb as described by Yang et al.,5 The limb was extended at an angle of 110° before casting as previously described.14,15 Consequently, the extensor digitorum longus (EDL) was stretched when the animal walked on this limb. The contralateral hind limb served as the control to the extended one.
The rabbits had free access to pellet food and water. At the end of the 1-week experimental period, they were sacrificed with an overdose injection of ketamine-xylazine along with placement in a CO2 chamber. The experimental protocol was approved by the institutional animal care committee.
The masseter (superficial and deep parts), lateral pterygoid, and EDL of both hind limbs were dissected immediately after the animals were sacrificed. Three representative regions from the mid-belly of each muscle were sectioned into 1-cm square pieces, flash-frozen in isopentane, cooled in liquid nitrogen, and stored at -80℃ for later sectioning.
Each sample was serially sectioned transversely at 8 µm on a cryostat at -20℃. Twenty-four adjacent serial sections of each muscle were divided in 4 sets of 3 slides. Two sections were mounted per slide. Each set of slides was stained with a specific MyHC antibody.
Immunostaining was accomplished as previously described16 with mouse monoclonal antibodies against slow, fast, embryonic, and neonatal MyHCs (Leica Bio systems, Newcastle, UK) on 4 consecutive sections to identify the expression of different MyHCs. For fiber composition analysis, the slides were visualized under a Light microscope (Eclipse, E600; Nikon, Tokyo, Japan). One section per muscle from each animal for each antibody was photographed under the same magnification by using a microscope digital camera (Spot RT; SPOT Imaging Soultions, Diagnositc Instruments Inc., Sterling Heights, MI, USA). Images were enhanced for contrast by using Adobe Photoshop®, and a single random area with approximately 150 fibers from each slide was selected and the percentage of immunopositive fibers was determined.
RT-PCR assay was performed on extracted total RNA by using TRIzol® (Invitrogen, Life Technologies, Carlsbad, CA, USA) to investigate the mRNA level differences between the control and the stretched muscles. Sequences for oligonucleotide primers were adapted from previous publications.17
Western blot assay was performed to confirm the phenotypic changes in the muscle fibers by using mouse monoclonal antibodies to sarcoplasmic reticulum Ca2+ ATPase 1 (SERCA1; Enzo Life Sciences Inc., Farmingdale, NY, USA), which is exclusively expressed in fast fibers, and SERCA2a (Enzo Life Sciences), which is exclusively expressed in slow fibers.18
The RT-PCR and western blot assays were repeated thrice by using muscle blocks obtained from 3 different rabbits.
Only immunohistochemical data were statistically analyzed. Inference on comparisons (8 extended vs. 8 control hind limbs) was tested by using Student's t-test with the SPSS 12.0 software program (IBM-SPSS, Chicago, IL, USA). The Kruskal-Wallis test was used for comparisons of the masticatory muscles (5 experimental vs. 3 control animals). Any measurement could not assume a distribution of measurements, for instance, embryonic MyHC concentration on the EDL, Kruskal-Wallis test was used for this variable. Differences were considered significant when p < 0.05.
Despite the presence of the intraoral appliance, no significant difference in body weight was observed between the control and the experimental groups during the experimental period. Both groups of rabbits lost less than 0.22 kg on the first day after the appliance and/or cast placement. By the third day, their weight returned to the baseline level and was maintained until sacrifice.
The RT-PCR assay showed marked differences in the mRNA expression of MyHC Iα and IIb in the stretched EDL (Figure 2A). MyHC Iα expression was noted only in the stretched condition. The stretched EDL had approximately double the amount of fibers expressing MyHC I compared with the control EDL (24.7 ± 3.54% vs. 11.7 ± 2.12%; p < 0.004; Figure 3A). A significant increase in the percentage of fibers stained positively for neonatal MyHC was also observed (20.7 ± 2.33% vs. 3.7 ± 1.46%; p < 0.001; Figure 3B). Moreover, a small but nonsignificant increase in the percentage of fibers expressing embryonic MyHC was detected in the stretched EDL (3.7% vs. 0%). The stretched and control limb muscles did not show significant differences in fast fibers.
The masticatory muscles responded differently to the stretch stimulus. The superficial and deep parts of the stretched masseter had increased mRNA expression of MyHC IIb and Iβ, respectively (Figure 2A), but similar proportions of slow and fast fibers (Figure 3C and D). Most massetric fibers in the control group stained positively for fast MyHC (Figure 3A). In the experimental group, a slight decrease in the percentage of fibers stained positively for slow MyHC was observed in the superficial (36.7% experimental vs. 40.6% control) but not the deep (44.7% experimental vs. 38.7% control) massetric part. However, these differences were not significant. Further, a very small percentage of fibers in the superficial (1.7%) and deep (0%) parts of the stretched masseter stained positively for embryonic MyHC; the superficial and deep parts of the control masseter had about 9.3% and 4.3% positively stained fibers, respectively, which were not significantly different from the percentages in the experimental animals. Moreover, the superficial and deep massetric parts in the experimental and control groups did not have significantly different percentages of fibers stained positively for neonatal MyHC.
As in the case of the deep massetric part, MyHC Iβ mRNA expression increased in the stretched lateral pterygoid (Figure 2A). This observation was supported by a significant increase in SERCA2a expression in the deep massetric part and lateral pterygoid (Figure 2B). The superficial massetric part did not show a significant difference in SERCA levels. The lateral pterygoid had mostly fast fibers: the percentages in the experimental and control groups were 84.5% and 77.1%, respectively, which were not significantly different (Figure 3E). Both groups had approximately 25% slow fibers in this muscle, without a significant difference. Because only the experimental group had lateral pterygoid fibers stained positively for neonatal MyHC (19.1%), the difference could not be tested statistically. No lateral pterygoid fiber in both groups stained positively for embryonic MyHC.
The western blot findings regarding the phenotypic changes in fibers from the stretched EDL are in agreement with those of previous studies,1,4,5 thus validating the immunohistochemical and RT-PCR assays. In particular, the significant increase in the percentage of MyHC I-expressing fibers in the stretched EDL well concerts with the RT-PCR result of decreased mRNA expression of MyHC IIb, indicating reduced transcriptional activity of MyHC IIb. The extended hind limb had a twofold increase in the percentage of fibers stained positively for slow MyHC, which was probably primarily MyHC Iα, implying a change from fast fibers to slow fibers in the stretched EDL. A previous study19 suggested an increase in the number of hybrid fibers expressing a combination of different MyHCs; however, the present results can be explained by an increase in the percentage of MyHC I-expressing fibers among hybrid fibers as the percentage of MyHC II-expressing fibers remained unchanged. Another explanation is that the increase or decrease in mRNA expression of MyHCs may not be sufficient to reflect changes in protein levels.
Although the molecular mechanism underlying fiber transition from a fast to a slow phenotype is presently not fully understood, a change in the synthesis or degradation of contractile proteins is a possible mechanism. According to Loughna et al.,15 passive stretch increases protein synthesis and has little or no effect on protein degradation. This may explain the lack of a change in the percentage of fibers expressing MyHC II and increase in the percentage of fibers expressing MyHC I.
Developmental (embryonic and neonatal) MyHCs usually emerge during the first postnatal week in many fibers.20 As the rabbit grows, these fibers are replaced with a definite MyHC-expressing fiber type in accordance with the muscle function, and are almost absent in the adult rabbit.21 However, the percentage of neonatal MyHC-expressing fibers increased when the rabbit EDL was stretched for a week in a previous study as well as in the present one. According to Yang et al.,5 this might be the result of stretch-induced muscle damage; he suggested that new muscle cells, generated from satellite cells, re-express their developmental program, including the expression of neonatal MyHC, which will later be replaced by the adult slow type. Jacobs-El et al.21 also suggested that the phenotypic change from fast to slow fibers involves the re-induction of the development program. Contraction of muscle in the stretched state, namely lengthening (or eccentric) contraction, often results in tissue damage. Satellite cells22,23 participate when postmitotic muscle tissue is injured. In the current study, a significant increase in the percentage of neonatal MyHC-expressing fibers was observed in the stretched EDL, which may indicate the involvement of satellite cells in a repair process. Damage from mild eccentric contractions, however, tends to recover in 2 weeks.24 Therefore, despite potential damage to the masticatory muscles, orthodontists may prefer to use functional appliances for gradual advancement and increase in the vertical dimension of the mandible to pro mote clinical outcomes.25
Numerous studies have shown differences between the limb and the masticatory muscles.26-28 One of the most studied masticatory muscles is the masseter. Unlike the limb muscles, the masseter has many hybrid fibers, which makes classification difficult.28,29 The rabbit masseter is divided into 3 compartments: superficial, intermediate, and deep. These compartments are easily distinguishable by their fiber orientation; however, tissue harvested from both the superficial and the deep parts was used in this study to avoid potential ambiguity. The masseter of adults in many species is composed of slow, alpha cardiac, IIa, IIx, and IIb fibers as well as developmental fibers such as embryonic and neonatal MyHC-expressing fibers.27,30 Widmer et al.31 demonstrated that MyHC subtypes vary ac cording to the location as follows: MyHC IIa-containing fibers are found mostly in the anterior and ventral regions, and MyHC IIb-expressing fibers are located in the posterior and dorsal regions of the masseter in mice. Both the superficial and the deep parts of the masseter had similar changes in the present study.
The experimental and control groups showed no significant differences in the percentages of slow and fast fibers in the masticatory muscles. This is in agreement with the study by Ohnuki et al.,9 who used a bite appliance to increase the vertical dimension in guinea pigs and found no difference in the expression of MyHC subtypes in the superficial part of the masseter between the control and the experimental groups. However, direct comparison of the data from the Ohnuki et al.9 study with those of the present study is difficult because their appliance was designed only to open and not protrude the mandible. In addition, the diet of the guinea pigs was changed to a soft diet, which may itself be a confounding factor. In a different study, Ohnuki et al.32 used a similar appliance to the present one in rats and found a significant difference in mRNA levels of MyHC in the superficial part of the masseter between the experimental and the control groups. In particular, they found an increase in the mRNA expression of MyHC IIa compared with MyHC IIb in the rats with the bite-opening appliance. This finding is consistent with the findings of Goldspink et al. and other groups,1,4,5 who found that stretch stimuli induce changes in MyHC expression along a continuum from fast fibers to slow fibers (i.e., IIb → IIx → IIa → I). Ohnuki et al.'s most recent report33 attempted to correlate the results of electromyograms (EMG) with sodium dodecyl sulfate polyacrylamide gel electrophoresis results that were not precisely quantitative. Moreover, they did not compare the masticatory muscles with the EDL in their studies on both rats and guinea pigs. Although the present study using rabbits was not performed for a sufficiently long time to assert the lack of changes in the masticatory muscles, as already discussed, significant changes in the EDL occurred in 7 days. In contrast, no significant changes occurred in the masticatory muscles.
Which factors would be responsible for the differences in the response to stretch between the limb and the masticatory muscles? One could speculate that the changes in the EDL were induced by compensatory hyperactivity in the control limb following immobilization of the extended limb. However, disuse often results in a transition from slow fibers to fast fibers, which would reduce the differences between the experimental and the control animals. Other reasons for the lack of significant changes in the stretched masticatory muscles could be the small sample size and the variation in the masticatory muscles did not allow detection of a significant increase in the percentage of fibers expressing slow MyHC.
In addition, the appliance may have been placed for an insufficient duration to induce a change to a higher percentage of fibers expressing MyHC I. Only one study has shown a change from type IIa to type I fibers in the masseter after 28 days of mandibular protrusion in pigs.11 The present findings very likely reflect fundamental temporal differences in the response to stretch of masticatory muscles compared with limb muscles. This view is supported in a recent review article stating that masticatory muscles do not seem to follow this strict pattern of fiber transition.34 Nevertheless, the RT-PCR and western blot results, although qualitative, suggest that the stretch stimulus by using the inclined-plane appliance may have altered the concentration of Ca2+-regulatory membrane proteins and MyHC mRNAs toward slow fiber phenotypes; however, the actual translation process for MyHCs did not occur in the deep part of the masseter and lateral pterygoid.
Few studies have shown the expression of developmental MyHCs in the masseter. Contrary to limb muscles, where developmental MyHC-expressing fibers disappear in the adult, Widmer et al.31 localized these fibers in the masseter, albeit in a small number (< 1%). In the present study, a few developmental MyHC-expressing fibers were found in the masseter and most of these were of the neonatal type, although some embryonic MyHC-expressing fibers were also found. To date, no other animal study has demonstrated changes in these fiber types in the masticatory muscles following the use of a bite-opening or protrusive appliance.
Data on the normal fiber composition of the lateral pterygoid is scarce. Easton and Carlson11 reported that the percentage of slow fibers in this muscle is 1% in rats. They also reported that the ratio of IIa-to-IIb fibers is 60:40. In the present study, 77% of the fibers expressed MyHC II and 25% showed MyHC I staining in the control rabbits. Further, they found an increase in the number of type I fibers and a 25% reduction in the number of IIb fibers after using a mandibular protrusive appliance in rats.11 The shortening of the lateral pterygoid potentially reverses the transition of fiber types observed in the passive stretch model,14 because shortening or contraction of the lateral pterygoid would occur as the mandible is protruded downward and forward. In the present study, a significant difference in fiber types was not found between the experimental and the control groups except for the neonatal MyHC-expressing fibers, which increased in percentage (from 0% to 19%) in the experimental group. However, increased mRNA expression of MyHC Iα and Iβ suggests hyperactivity of the corresponding muscle and increased SERCA2a expression suggest a behavior in which calcium ions favor slow fibers.
Fast-to-slow fiber transition in the stretched masticatory muscles was not observed in the present study. Although others have suggested that this change also occurs in the masticatory muscles, the current findings do not support this view completely. Changes in the masseter and lateral pterygoid are possibly more subtle than those in the limb muscles or take longer time to occur because of anatomical and functional differences. One of the reasons perhaps is that the masticatory muscles are predominantly fast muscles but contain a greater number of hybrid fibers than the EDL.35 Functional appliances for correction of malocclusions invariably stretch jaw closers; however, their efficacy remains highly controversial. The slow transition of fiber types in the masticatory muscles may be a reason why orthodontists favor the long-term use of functional appliances.
The results of this study confirm the findings of others suggesting a change in the MyHC composition of fibers in stretched limb muscles, providing confidence in the present methodology. On the other hand, the insufficient sample size does not eliminate type 2 errors. An increase in the amount of fibers expressing neonatal MyHC in stretched limb muscles may also suggest reprogramming of new satellite cells that respond to local tissue damage, which may occur because of the stretch.22 The same fiber changes in the stretched masticatory muscles were not observed in this study. Considering the results of the RT-PCR and western blot assays, however, a prolonged stretch stimulus would induce a detectable change to a slow fiber phenotype in the deep part of the masseter and lateral pterygoid.
1. Changes in fiber composition of masticatory muscles may take longer time to occur compared to those of limb muscles. This slow conversion speed may be a reason for the long-term use of a functional appliance that is favored by orthodontists.
2. Significant mRNA changes without large changes in MyHC protein composition may be an adaptive response for homeostasis frequently observed in a skeletal muscle with a more complex structure such as masticatory muscles.
Figures and Tables
Figure 1
Muscles subjected to a stretch stimulus. A, The extensor digitorum longus is found in the lower part of the hind limb. B, The deep and superficial parts of the masseter have distinctive patterns of fiber orientation; yet, both muscles stretch when the animal bites.
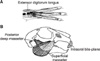
Figure 2
Results of the RT-PCR and western blot assays. A, The mRNA expressions of different MyHCs detected by RT-PCR assay of the stretched (+) and control (-) muscles are shown. β-actin was used as an internal control. B, The SERCA1 and SERCA2a expression levels in the deep part of the masseter and lateral pterygoid assessed by western blot assay are shown. The graph denotes the relative increase in SERCA expression intensity when the muscles were stretched. EDL, Extensor digitorum longus; MyHC, myosin heavy chain; SERCA, sarcoplasmic reticulum Ca2+ ATPase.
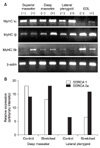
Notes
References
1. Goldspink G, Scutt A, Loughna PT, Wells DJ, Jaenicke T, Gerlach GF. Gene expression in skeletal muscle in response to stretch and force generation. Am J Physiol. 1992. 262:R356–R363.


2. Goldspink G. Changes in muscle mass and phenotype and the expression of autocrine and systemic growth factors by muscle in response to stretch and overload. J Anat. 1999. 194:323–334.


3. Pattullo MC, Cotter MA, Cameron NE, Barry JA. Effects of lengthened immobilization on functional and histochemical properties of rabbit tibialis anterior muscle. Exp Physiol. 1992. 77:433–442.


4. Williams P, Watt P, Bicik V, Goldspink G. Effect of stretch combined with electrical stimulation on the type of sarcomeres produced at the ends of muscle fibers. Exp Neurol. 1986. 93:500–509.


5. Yang H, Alnaqeeb M, Simpson H, Goldspink G. Changes in muscle fibre type, muscle mass and IGF-I gene expression in rabbit skeletal muscle subjected to stretch. J Anat. 1997. 190:613–622.


6. Pette D, Staron RS. Cellular and molecular diversities of mammalian skeletal muscle fibers. Rev Physiol Biochem Pharmacol. 1990. 116:1–76.


7. Pette D, Staron RS. Myosin isoforms, muscle fiber types, and transitions. Microsc Res Tech. 2000. 50:500–509.


8. Essig DA, Devol DL, Bechtel PJ, Trannel TJ. Expression of embryonic myosin heavy chain mRNA in stretched adult chicken skeletal muscle. Am J Physiol. 1991. 260:C1325–C1331.


9. Ohnuki Y, Saeki Y, Yamane A, Kawasaki K, Yanagisawa K. Adaptation of guinea-pig superficial masseter muscle to an increase in occlusal vertical dimension. Arch Oral Biol. 1999. 44:329–335.


10. Gedrange T, Luck O, Hesske G, Büttner C, Seibel P, Harzer W. Differential expression of myosin heavy-chain mRNA in muscles of mastication during functional advancement of the mandible in pigs. Arch Oral Biol. 2001. 46:215–220.


11. Easton JW, Carlson DS. Adaptation of the lateral pterygoid and superficial masseter muscles to mandibular protrusion in the rat. Am J Orthod Dentofacial Orthop. 1990. 97:149–158.


12. Sfondrini G, Reggiani C, Gandini P, Bovenzi R, Pellegrino MA. Adaptations of masticatory muscles to a hyperpropulsive appliance in the rat. Am J Orthod Dentofacial Orthop. 1996. 110:612–617.


13. Petrovic A, Stutzmann J, Oudet C. McNamara JA, Carlson DS, Ribbens KA, editors. Defects in mandibular growth resulting from condylectomy and resection of the pterygoid and masseter muscles. The effect of surgical intervention on craniofacial growth, Mono graph 12, Craniofacial growth series. 1982. Ann Arbor: Center for Human Growth and Development.
14. Loughna PT, Izumo S, Goldspink G, Nadal-Ginard B. Disuse and passive stretch cause rapid alterations in expression of developmental and adult contractile protein genes in skeletal muscle. Development. 1990. 109:217–223.


15. Loughna P, Goldspink G, Goldspink DF. Effect of inactivity and passive stretch on protein turnover in phasic and postural rat muscles. J Appl Physiol. 1986. 61:173–179.


16. Pae EK, Hyatt JP, Wu J, Chien P. Short-term electrical stimulation alters tongue muscle fibre type composition. Arch Oral Biol. 2007. 52:544–551.


17. Jaschinski F, Schuler M, Peuker H, Pette D. Changes in myosin heavy chain mRNA and protein isoforms of rat muscle during forced contractile activity. Am J Physiol. 1998. 274:C365–C370.
18. Eizema K, van der Wal DE, van den Burg MM, de Jonge HW, Everts ME. Differential expression of calcineurin and SR Ca2+ handling proteins in equine muscle fibers during early postnatal growth. J Histochem Cytochem. 2007. 55:247–254.


19. Hori A, Ishihara A, Kobayashi S, Ibata Y. Immunohistochemical classification of skeletal muscle fibers. Acta Histochem Cytochem. 1998. 31:375–384.


20. Bredman JJ, Weijs WA, Korfage HA, Brugman P, Moorman AF. Myosin heavy chain expression in rabbit masseter muscle during postnatal development. J Anat. 1992. 180:263–274.
21. Jacobs-El J, Zhou MY, Russell B. MRF4, Myf-5, and myogenin mRNAs in the adaptive responses of mature rat muscle. Am J Physiol. 1995. 268:C1045–C1052.


22. Rathbone CR, Wenke JC, Warren GL, Armstrong RB. Importance of satellite cells in the strength recovery after eccentric contraction-induced muscle injury. Am J Physiol Regul Integr Comp Physiol. 2003. 285:R1490–R1495.


23. Hill M, Goldspink G. Expression and splicing of the insulin-like growth factor gene in rodent muscle is associated with muscle satellite (stem) cell activation following local tissue damage. J Physiol. 2003. 549:409–418.


24. Chen HL, Nosaka K, Chen TC. Muscle damage protection by low-intensity eccentric contractions remains for 2 weeks but not 3 weeks. Eur J Appl Physiol. 2012. 112:555–565.


25. Hägg U, Rabie AB, Bendeus M, Wong RW, Wey MC, Du X, et al. Condylar growth and mandibular positioning with stepwise vs maximum advancement. Am J Orthod Dentofacial Orthop. 2008. 134:525–536.


26. Bredman JJ, Wessels A, Weijs WA, Korfage JA, Soffers CA, Moorman AF. Demonstration of 'cardiac-specific' myosin heavy chain in masticatory muscles of human and rabbit. Histochem J. 1991. 23:160–170.


27. Bredman JJ, Weijs WA, Moorman AF, Brugman P. Histochemical and functional fibre typing of the rabbit masseter muscle. J Anat. 1990. 168:31–47.
28. Rowlerson A, Pope B, Murray J, Whalen RB, Weeds AG. A novel myosin present in cat jaw-closing muscles. J Muscle Res Cell Motil. 2000. 2:415–438.


29. Sciote JJ, Morris TJ. Skeletal muscle function and fibre types: the relationship between occlusal function and the phenotype of jaw-closing muscles in human. J Orthod. 2000. 27:15–30.


30. Horton MJ, Brandon CA, Morris TJ, Braun TW, Yaw KM, Sciote JJ. Abundant expression of myosin heavy-chain IIB RNA in a subset of human masseter muscle fibres. Arch Oral Biol. 2001. 46:1039–1050.


31. Widmer CG, Morris-Wiman JA, Nekula C. Spatial distribution of myosin heavy-chain isoforms in mouse masseter. J Dent Res. 2002. 81:33–38.


32. Ohnuki Y, Saeki Y, Yamane A, Yanagisawa K. Quantitative changes in the mRNA for contractile proteins and metabolic enzymes in masseter muscle of bite-opened rats. Arch Oral Biol. 2000. 45:1025–1032.


33. Ohnuki Y, Kawai N, Tanaka E, Langenbach GE, Tanne K, Saeki Y. Effects of increased occlusal vertical dimension on daily activity and myosin heavy chain composition in rat jaw muscle. Arch Oral Biol. 2009. 54:783–789.

